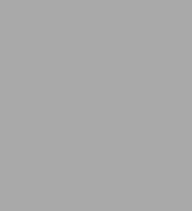
Chemical Oxidation Applications for Industrial Wastewaters
360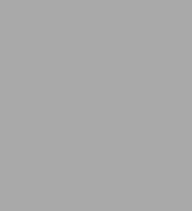
Chemical Oxidation Applications for Industrial Wastewaters
360Paperback
-
PICK UP IN STORECheck Availability at Nearby Stores
Available within 2 business hours
Related collections and offers
Overview
Chemical Oxidation Applications for Industrial Wastewaters is an essential reference for lecturers, researchers, industrial and environmental engineers and practitioners working in the field of environmental science and engineering.
Visit the IWA WaterWiki to read and share material related to this title: http://www.iwawaterwiki.org/xwiki/bin/view/Articles/CHEMICALOXIDATIONAPPLICATIONSFORINDUSTRIALWASTEWATERS
Authors: Professor Olcay Tünay, Professor Isik Kabdasli, Associate Professor Idil Arslan-Alaton and Assistant Professor Tugba Ölmez-Hanci, Environmental Engineering Department, Istanbul Technical University, Turkey.
Product Details
ISBN-13: | 9781843393078 |
---|---|
Publisher: | IWA Publishing |
Publication date: | 10/12/2010 |
Pages: | 360 |
Product dimensions: | 6.12(w) x 9.25(h) x 0.75(d) |
Read an Excerpt
CHAPTER 1
Introduction to redox reactions
1.1 INTRODUCTION
1.1.1 Redox processes
Chemical oxidation is a process in which the oxidation state of an atom is increased. The atom being oxidised may be in the elemental form or in a substance like a molecule or ion. The term "oxidised" is also used for the substance containing the oxidised atom. If the oxidation takes place within biological processes the terms biological or biologically-mediated oxidation are used. Chemical reduction is the process by which the oxidation state, the valence, of an atom is reduced. Every oxidation reaction is accompanied by a reduction reaction and these reactions are termed redox reactions.
For inorganic redox reactions, oxidation and reduction are brought about by electron transfer. Oxidation is the loss of electrons and reduction is the gain of electrons by an atom. In the below example:
S2- + I2 -> 2I- + S0 (1.1)
Sulphide ion is oxidised to elemental sulphur by losing two electrons, while elemental iodine is reduced to iodide ion by gaining two electrons. In the organic reactions, the mechanism is more complex. An organic reaction oxidation is carried out by replacement of one of the electrons making up the covalent bond between two atoms, by changing one of the atoms in a way for reversing the order of electronegativities of the atoms. If atoms A and B are tied up with a covalent bond and atom A is more electronegative than atom B, replacement of atom B by atom C which is more electronegative than atom A, through breaking the A-B bond and formation of an A-C bond, results in the oxidation of atom A. The basis of this process is expressed by the Pauling rule stating that "In a covalent bond the charge of an atom is found by assigning each shared pair of electrons completely to the more electronegative of the two atoms sharing them. An electron pair shared by two atoms of the same electronegativity is split between them" (Stumm and Morgan 1996).
1.1.2 Thermodynamics of redox reactions
A redox reaction is represented by two half-reactions; oxidation and reduction. This representation is quite useful in analysing the redox reactions and defining thermodynamic aspects of the reactions. The half-reactions are "coupled". The redox reaction:
I2(aq) + 2S2O2-3 -> 2I- + S4O2-6 (1.2)
is represented by the following half-reactions:
reduction: I2 (aq) + 2e- -> 2I- (1.3)
oxidation 2S2O2-3 -> S4O2-6 + 2e- (1.4)
In the reduction half-reaction the reactant that accepts electrons is termed "oxidant". In the oxidation half-reaction the reactant that donates electrons is termed "reductant". An important definition in redox stoichiometry is "equivalent weight". Equivalent weight is calculated by dividing the formula weights of reductant and oxidant to number of electrons in the half-reactions. In thermodynamics spontaneity of a reaction occurring under constant pressure is determined using Gibbs free energy. The negative free energy change indicates that the redox reaction is spontaneous in the direction it is written. The same approach is applied to half-reactions.
As indicated above half-reactions are hypothetical presentations. A half-reaction cannot occur unless it is combined with another half-reaction to yield a redox reaction provided that the redox reaction is thermodynamically possible. So the two half-reactions are "coupled". They are coupled because the free electrons cannot exist in solution and the electrons given off in a half-reaction must be received by a substance taking part in the second half-reaction. On the other hand, they are energetically coupled in that the spontaneity of the reactions is judged by the value of the free energy change of final redox reaction. Free energy change of any half-reaction may be positive indicating it is not spontaneous, but the half-reaction proceeds as long as free energy change of the redox reaction is negative, since the half-reactions are energetically coupled. Electron transfer between the substances is readily carried out in solution. Now assume that the half-reactions (Eq. 1.3) and (Eq. 1.4) are attempted to be realised in two separate containers marked I and II in Figure 1.1. None of the half-reactions, even the one having negative free energy can occur. If electron transfer is provided through electrodes dipped in both containers and they are connected with a conductor wire, the half-reactions still do not occur, because electroneutrality condition cannot be satisfied in both containers. In Container I the lack of electrons causes positive charge accumulation and in Container II negative charges will accumulate. If we provide ion transfer between the containers electroneutrality is satisfied and half-reactions begin to take place and electrons flow through the connecting wire. Electron flow creates electric current whose energy corresponds to free energy change of the total redox reaction. Such a system is named as an electrochemical cell.
An electrochemical cell (or galvanic cell) is a device for producing an electric current (potential difference) as a result of electrochemical (redox) reactions. A cell consists of two electrodes, an electrolyte (electrolytic conductor) in which electrodes are immersed and a metal conductor by which electrodes are connected. Metal conductor and electrolyte are also called as external circuit and internal circuit, respectively. The potential difference of the electric current obtained by the operation of the cell is (more precisely the maximum potential difference during reversible operation of the cell) called the electromotive force (e.m.f.) The e.m.f. of a cell is equal to the algebraic sum of the potential differences (jumps) at the interfacial boundaries (surfaces of electrodes) of the cell. e.m.f. of a cell is denoted as Ecell, and is defined, in a similar manner to reaction free energy, as:
Ecell = Eox + Ered (1.5)
where Eox and Ered denote potentials of oxidation and reduction half-reactions, respectively. These potentials are termed electrode potentials of the half-reactions. Electrode potential of a half-reaction is a characteristic of the reaction and related to the free energy of this half-reaction.
A cell produces electricity on its external circuit. This corresponds to the work by the cell on the surroundings. Electrical work is defined as:
-wel = Q × E (1.6)
where wel is the electrical work and Q is the charge which is moved across a potential difference E. Negative sign of wel is due to the convention that the work (energy) transferred to surroundings by the system is taken negative. The charge Q is carried by the electrons. Faraday constant (F) expresses the charge of a mole of electrons and equals 96,490 Coulomb per mole of electrons. n denotes the number of moles of electrons per mole of reaction with the unit (mol/mol). Then total charge, Q, passing through the external circuit is:
Q = n × F (1.7)
The electrical work is, therefore:
-wel = n × F × E (1.8)
On the other hand, the non-expansion work of a reversible electrochemical cell, in other words ΔG equals wel, then:
ΔG = -n × F × E (1.9)
is the equation that relates free energy to the e.m.f. of the cell, or electrode potential of half-reactions. E is measured in volts. Since:
Joule = Coulomb × volt (1.10)
the units of ΔG are J/mol, or if two sides of Eq. (1.9) are divided by 4.18 (this is made by assuming that the Faraday constant as 23,060) in cal/mol.
Since individual half-cell reactions do not occur, absolute value of a half-cell potential cannot be determined. However, a half-cell potential can be measured in relation to the potentials of other half-cells. This leads to adopting a reference half-cell reaction and determining the potentials of all other half-reactions against this reference half-reaction. For this purpose, the hydrogen half-cell has been adopted universally as the reference cell. The half-cell reaction:
H+ (aq) + e- -> 1/2 H2 (g) (1.11)
is assigned the standard potential of 0.000 V under standard conditions (1atm H2 pressure, 25°C and pH = 0). This assignment is consistent with ΔGof = 0 for H2(g) and H+(aq). The hydrogen reference electrode under standard conditions is named standard hydrogen electrode and abbreviated SHE. For the cell formed by the below reactions where Eo indicates standard conditions:
[MATHEMATICAL EXPRESSION OMITTED] (1.12)
[MATHEMATICAL EXPRESSION OMITTED] (1.13)
Eocell is determined by summation of the half-reactions as:
[MATHEMATICAL EXPRESSION OMITTED] (1.14)
and:
[MATHEMATICAL EXPRESSION OMITTED] (1.15)
by definition [MATHEMATICAL EXPRESSION OMITTED] and:
[MATHEMATICAL EXPRESSION OMITTED] (1.16)
Electrode potential of a half-reaction determined against hydrogen reference electrode, under standard conditions, is termed standard electrode potential. In the above given example Eocell is the standard electrode potential of half-reaction [MATHEMATICAL EXPRESSION OMITTED]. Direction of the reaction is important for the expression of the electrode potential. Standard electrode potential is universally adopted to be the potential of a half-reaction written in the direction of reduction. Since [MATHEMATICAL EXPRESSION OMITTED] half-reaction is written in the direction of reduction the value of [MATHEMATICAL EXPRESSION OMITTED] is its standard electrode potential. Electrode potentials of some reactions are tabulated in Table 1.1. The superscript in the symbol Eo denotes that all the reactions are at standard state. Standard state indicates that reactions are at 1 atm pressure, 25°C temperature while all reactants and products in the reactions in unit activity or 1 molar where it is applicable.
The E value of a reaction is independent of reaction stoichiometry, however, as Eq. (1.9) indicates; the ?G value is dependent on the stoichiometry. ΔG is an extensive property and its value depends on the amount of the matter. Therefore, ΔG values of reactions are always additives. E value of an oxidation reduction reaction cannot be found by the addition of the E values of the half-reactions yielding the resulting oxidation reduction reaction. E value is an intensive property so is independent of the amount of matter. The simple addition of E values of the half-reaction is justified only for the case where number of electrons in the half-reactions is equal and cancels each other resulting in a cell reaction.
In Eq. (1.9), F and n are positive entities; therefore ?G and E have reverse signs. A reaction is spontaneous when ?G is a negative number. Then, redox reactions are spontaneous as their E values are positive, in other words, as they make up a cell and produce electricity. According to this thermodynamic property in actual conditions, the half-reactions determine their direction in order to yield a redox reaction with positive E value. The half-reaction with higher E value has the tendency to be reduced. Then, in a redox reaction, the half-reaction with higher E is a reduction while the one with smaller E is oxidation. That is why the substance associated with higher E value is a stronger oxidant with respect to one with smaller E value. The value of ΔG for any condition other than standard conditions is known to be found using:
ΔG = ΔGo + R × T × lnQ (1.17)
where R is ideal gas constant (8.31 J/mol.K), T is temperature (in K), and lnQ is the reaction ratio. For the general equation:
aA + bB -> CC + dD (1.18)
Q is given by the equation:
Q = {C}c{D}d/{A}a{B}b (1.19)
The activities in Eq. (1.19) may be replaced with molar concentrations, if the solution is diluted. If all terms of Eq. (1.17) is divided by (-n×F) the equation:
E = Eo RT/nF × lnQ (1.20)
is obtained. This equation is known as Nernst equation. At 25°C Nernst equation converts to:
E = Eo - 0.059/n × log Q (1.21)
and at equilibrium ΔG = 0 and E = 0:
Eo = RT/nF × lnK (1.22)
is obtained. In Eq. (1.22) K is equilibrium constant.
Another useful parameter pε is defined as:
pε = -log{e-} (1.23)
and expresses the electron activity of a solution at equilibrium. pε is the hypothetical tendency of a solution to accept or to donate electrons, analogous to pH. In a reducing solution electron activity is high, and pε is low, indicating high tendency to donate electrons. In an oxidising solution, electron activity is low and pε is high indicating high tendency to accept electrons.
The equations developed above help to understand and bring solutions to redox reactions. Another way of evaluating the redox data is to use diagrams derived through the basic equations and related thermodynamic data. These diagrams serve to assimilate the system behaviour at a glance. There are several types of diagrams designed for specific purposes. Latimer diagrams show half-cell potentials of an element and provide ease of evaluation for complex systems such as the elements acquiring several oxidation numbers. Free energy-oxidation state diagrams (Frost diagrams) are similar to Latimer diagrams, but are based on free energy changes relative to a common reference point. Potential-pH diagrams are prepared using the Nernst equation and are quite useful for redox evaluations for varying pH. Thermodynamically most stable form of an element at a given pH and electrode potential can be assessed using predominance-area (Pourbaix) diagrams.
An electrochemical cell is a device that produces electric current as a result of a redox reaction. Therefore, an electrochemical cell converts chemical energy to electrical energy. Electrolysis is a reverse process in which redox reactions proceed under the action of an electric current. Since the work obtained from an isothermal reaction is maximum when the reaction is carried out under reversible conditions, the electric work that is obtained from an electrochemical cell is maximum when the cell is operating under reversible conditions. Reversible cells provide maximum work and all thermodynamic expressions developed in this section apply to reversible cells. An electrochemical cell is regarded as reversible if a small amount of current can be passed in either direction without appreciable effect on the measured potential. In a cell as given in Figure 1.1 the electrode to which anions migrate to satisfy the electroneutrality condition is named as anode (positive electrode). The anode releases electrons into external circuit, therefore, it is the place where oxidation half-reaction takes place. The cathode (negative electrode) on the contrary, receives electrons from external circuit by which reduction half-reaction occurs. There are several types of electrodes. The metal electrode reacts reversibly with its cations in the electrolyte. These electrodes are reversible relative to the cations and called electrodes of the first kind. The hydrogen electrode belongs to this kind and it is also a gas electrode. Electrodes of the second kind are the metal electrodes covered by a layer of sparingly soluble salt of this metal and they are in a solution saturated with this salt and containing a highly soluble salt with the same anion. This kind of electrodes is reversible with respect to cation and anion but only the concentration of anion can be controlled. The oxidation-reduction electrodes are metals (noble metals) which exchange only electrons with the electrolyte.
(Continues…)
Excerpted from "Chemical Oxidation Applications for Industrial Wastewaters"
by .
Copyright © 2010 IWA Publishing.
Excerpted by permission of IWA Publishing.
All rights reserved. No part of this excerpt may be reproduced or reprinted without permission in writing from the publisher.
Excerpts are provided by Dial-A-Book Inc. solely for the personal use of visitors to this web site.