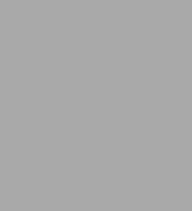
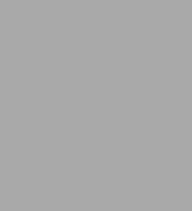
Hardcover(Edition. ed.)
-
PICK UP IN STORECheck Availability at Nearby Stores
Available within 2 business hours
Related collections and offers
Overview
About the Author
Read an Excerpt
Alternatives to Conventional Food Processing
By Andrew Proctor
The Royal Society of Chemistry
Copyright © 2011 The Royal Society of ChemistryAll rights reserved.
ISBN: 978-1-84973-037-2
CHAPTER 1
Introduction to Green Chemistry
JAMES H. CLARK
Green Chemistry Centre of Excellence, University of York, York, UK, YO10 3HW
1.1 Introduction
This brief chapter provides readers who are unfamiliar with 'green technology' with a broad understanding of 'green principles' to better appreciate the social, economic and technical context that necessitate the development of alternative food processing techniques, that reduce energy requirements and/or organic 'chemical' solvent use. Life-cycle analysis is also introduced as a key concept in evaluating the sustainability of any green technology that uses alternative fuels, or reduces energy use, relative to established technology. The issue of biofuels is explored and supercritical extraction briefly discussed as an example of green transformation.
Developing alternative technologies and products are essential to move the food industry, and other industries, towards sustainable processing and to reduce commercial energy use and thereby responsibly preserve local and global environments. This activity is called Green Chemistry, Green Engineering or Sustainable Design and requires input from various scientific, engineering, technological, environmental, economic and legal disciplines. It is influenced by multiple drivers which affect the creation of new green technologies, which are outlined in Figure 1.1.
Green chemistry/technology involves the sustainable manipulation of chemicals and materials to value-added products, and therefore involves both new processes and products. The principles of green chemistry were first outlined in the 1990's. However, it can also be considered as simply a means of maximizing the efficient use of resources and achieving cost savings, while minimizing negative human and environmental impact (Figure 1.2). Green chemistry requires new, low environment impact technologies to reduce energy use, facilitate greater use of catalysis and environmentally benign processing and avoidance of harmful organic solvents. Furthermore, it also involves reducing the number of processing steps in industrial manufacturing to obtain the same products in fewer processing steps with less energy and waste materials.
Green engineering thus requires the application of fundamental engineering concepts and practices to reduce the environmental impact of current manufacturing practices. The United States Environmental Protection Agency describes this as the design, commercialization and use of processes and products that are economically feasible to implement, while minimizing pollution production and human/environmental risk by incorporating the principles of process optimization, life cycle assessment and the environmental economics of processing.
Sustainable design differs from a conventional approach by including a consideration of the environmental impact on local and global ecosystems. The 'three pillars of sustainable development' have been described as society, ecology and environment, often referred to as the triple bottom line of true process sustainability (Figure 1.3). This eco-efficient tool developed by the world's largest chemical company, BASF, integrates these pillars and quantifies the most sustainable processes by including the health and safety costs. Ecological impacts are evaluated by life cycle assessment by considering land use, raw material consumption, atmospheric emissions and water-borne pollutants.
The situation is made more complex by resource allocation as described by the food versus fuel debate, or as more correctly stated the food verses fuel versus feed versus chemicals issue, which stimulated discussion regarding the use of agricultural land. However, this debate is often oversimplified, as will now be considered. As oil reserves are used and the demand from a growing population increases we may experience conflict in the use of resources. The use of oil imported for chemicals plastic production and transport fuels is non-sustainable and could have a serious negative environmental and societal impact if a more efficient use of energy and greater use of alternative renewable fuels from agriculture, and other resources, is not realized. However, the food versus fuel debate has increased awareness of the direct and indirect cost of crop production. Therefore, the environmental footprint of corn production for bioethanol must include the impact of agrichemicals used to manage the land and the cost of using more land to grow the food crops that the non-food use of corn has displaced. However, there is an overall benefit if displacing a largely exported food crop from a region resulted in more locally produced food being consumed. It is also important to take into account the environmental benefits of not using petroleum, including the avoidance of environmental damage at oil extraction sites. This potential for environmental damage will become greater as efforts are made to obtain less accessible petroleum sources, such as in deep water marine environments sources in the Gulf of Mexico, the Mediterranean Sea and the Canadian tar sands. The environmental cost of oil use in food production should also include the carbon footprint developed by food production that uses oil as an energy source at various life cycle stages. Nevertheless, there may be certain biofuel crops that can grow on land not suitable for non-food or non-feed crops, and therefore do not compete with food crops. Jatropha is an example of a biodiesel feedstock which can be grown on poor quality land. Sugar cane, which is grown in excess of local food needs, can be used for bioethanol production, thereby reducing oil use and supporting the local economy. Although sugars are readily converted to bioethanol, there is a much greater volume of lignocellulose from food and forestry production and processing which is a potential source of renewable carbon (Figure 1.4). Lignocellulose represents a second generation biofuel source, but there are significant technical challenges in cellulose conversion to bioethanol fuel that are currently being addressed.
Reducing the global environmental burden is not feasible by population control or by curbing the significant growth in world prosperity, which is driven largely by emerging economies, such as those of China, India, Brazil and Russia. However, some reduction in the global burden could be achieved by a) reduction in resource use i.e. dematerialization; and b) substitution of traditional resources with greener, more sustainable alternatives i.e. transmaterialization.
Dematerialization steps should be included throughout a product's lifecycle to increase process efficiency from the pre-manufacturing stage to a product's end-of life (Figure 1.5). The final stages of a product's life should include some recovery of environmental cost through reuse, recycling, or combustion for energy. For example, ash from combustion may be used as plant mineral nutrients or as useful products in themselves e.g. carbon and silicate adsorbents. In contrast, many manufacturing processes produce more waste than product, with the waste product ratio being > 100 for high value chemicals such as pharmaceuticals. Therefore, sustainable production and consumption based on traditional non-renewable resources is not reasonable.
The need for more efficient use of limited resources is well illustrated by the use of many mineral resources commonly used in consumer goods. The present rates of consumption of platinum, germanium, silver and zinc are not sustainable (Figure 1.6). An effective way to show the increasing demand on the worlds resources is to calculate the number of 'earth-like planets' necessary to sustain the lifestyles of various world regions. This was found to be four for the world's richest regions and less than one for the most underdeveloped regions, with a world average of two. The global disparity in resource utilization may play a role in affecting international social stability but may be reduced by dematerialization and transmaterialization technologies, i.e. 'one person's waste is another person's profit'. The key requirements of this idealized sustainable production life cycle are shown in Figure 1.7.
Transportation is an important part of lifecycle costs as unless it can be achieved at very low resource and environmental cost, it is not sustainable and limits geographical distribution of life-cycle stages.
1.2 Resources for Remanufacturing
Non-food biomass, agricultural waste, forestry co-products, grasses and other high volume high turn-over plants are recognized as having the greatest potential as a sustainable carbon source. Such biomass would provide many organic-based materials and would provide the equivalent to using about 20% of the world's oil supply.
Waste management legislation can be of assistance in the transmaterialization or 'cradle-to-cradle potential of waste use. For example, EU legislation on Waste Electronic and Electrical Equipment (WEEE) require the producer to take responsibility at the end-of-life. This is resulting in logistic development for WEEE collection and facilitation of collection of scarce inorganic chemicals from this resource. For example, GSK recycled PET in bottles to improve waste management for production of recycled soft drink bottles, Coca Cola is building a new factory for processing of waste plastic and Hotpoint is producing the backs of washing machines from recycled plastics. It is important that extraction of valuable components has a low environmental impact. A good example of this is the use of supercritical carbon dioxide to extract liquid crystal compounds from WEEE. LCD displays consist of complex mixture of organic compounds and their production has a substantial footprint due to the many steps in manufacture and the waste produced. Therefore, for LC recovery from WEEE to be environmentally and economically effective, uses need to be found for more than the organic chemicals that represent a small proportion of a modern display panel.
The 'E factor', devised by Roger Sheldon, is a useful first approximation of the environmental footprint and is expressed as the ratio of waste to product:
E = Waste produced throughout manufacturing process/Product
1.3 Case Studies: making the most of waste
1.3.1 Biofuels-Friend or Foe?
Interest in biofuels during the last decade has led to more recent concerns about using agricultural crops for fuel, due to a belief that this would result in increasing food prices.
However, there are several reasons why biofuels are attractive alternatives to fossil fuels.
1. Biofuels are derived from renewable resources; although crop cultivation and transport consume traditional fossil resources i.e. fertilisers, agricultural chemicals, fuels for agricultural and transport vehicles.
2. Biofuels can lead to a reduction in carbon emissions and hence climate change mitigation; Incomplete use of renewable resources at each stages of the biofuel lifecycle, suggest that carbon dioxide reduction may be less than anticipated.
3. Biofuels can help to increase farm income and contribute to rural development; many countries are beginning to use land to grow energy crops. For example, plans to grow sugar cane in Tanzania to reduce their dependence on foreign oil. Farmers in more prosperous region see energy crops as an alternative to traditional crops. Co-products from food crops such as straw and hulls, are being used as fuel products e.g. pelletized straw for co-firing with coal in electricity power stations.
4. Biofuels can improve energy security; reduced dependence on imported energy is a priority in many parts of the world.
The 'biorefinery principle' will be the means by which fuel, energy and value added products are obtained from biomass at a single integrated site. The various components and energy will be removed sequentially. Extracted starch, cellulose and lignin can be processed into various chemicals and materials. In contrast to the relatively stable composition of petroleum oil, there is compositional variation between species and production seasons. Therefore, there needs to be flexibility in processing conditions when dealing with various biomass materials. Alternatively, unprocessed biomass can be used in its natural form or slightly modified to produce useful materials.
1.3.2. Extraction of Chemicals and Food from Biomass
Isolation of valuable chemicals from biomass could potentially form the initial processing step of many future biorefineries. Although this already represents the first stage of all current oil-bearing crop food processing plants (e.g. oilseed crushing and solvent extraction) and green biorefineries (e.g. green grass pressing), this concept could be extended to the extraction of high value surface chemicals from many biomass types. Valuable wax products, for example, have been extracted from wheat straw using supercritical carbon dioxide, a green chemical technology that allows the production of many consumer products e.g. cosmetics, polishes, nutraceuticals, with no solvent residues. This book contains two chapters that exhaustively address the use of super-critical carbon dioxide by the food industry for extraction and pasteurization sterilization, respectively. The discussion below briefly examines the technology as an example of green processing.
Carbon dioxide can exist in the solid, liquid or gaseous phase. Furthermore, if the liquid phase is taken beyond the critical points of temperature and pressure, a supercritical fluid is formed, which in simple terms can be considered as a dense gas.
Liquid and supercritical carbon dioxide can be used as an effective extraction solvent. Liquid carbon dioxide behaves like a non-polar solvent, and preferentially dissolves non-polar compounds. This is a very useful property of carbon dioxide as its solvating power can be easily manipulated by altering pressure and temperature. Thus non-polar and slightly polar molecules, such as terpenes, can be soluble in liquid carbon dioxide. Supercritical carbon dioxide can be a good solvent for many pharmacologically or industrially valuable molecules, such as the antimalarial compound artemisinin. Even very polar compounds such as polyphenols can be extracted by supercritical carbon dioxide at high enough pressures and temperatures.
In a typical supercritical carbon dioxide extraction plant, carbon dioxide is pumped through columns containing the material to be extracted. What distinguishes liquid and supercritical carbon dioxide from solvents such as hexane and ethanol is that (i) the carbon dioxide solvent is released as a gas and recycled in the process, so that a solvent-free extract and solvent free extracted material is produced and (ii) the solvating power of carbon dioxide can be manipulated readily by temperature, pressure, flow rate and residence time modification. The extraction can therefore be highly selective, which greatly reduces the need for further downstream purification and refining.
The remainder of the book discusses the legal context of green food processing and important technologies that are being developed for industrial use to produce high quality food products. The sustainability, food quality, energy needs and technical limitations are discussed with an evaluation of how close each technology is to commercialization, if not already commercialized.
(Continues...)
Excerpted from Alternatives to Conventional Food Processing by Andrew Proctor. Copyright © 2011 The Royal Society of Chemistry. Excerpted by permission of The Royal Society of Chemistry.
All rights reserved. No part of this excerpt may be reproduced or reprinted without permission in writing from the publisher.
Excerpts are provided by Dial-A-Book Inc. solely for the personal use of visitors to this web site.