In 1944, the Nobel Prize–winning physicist Erwin Schrödinger published a groundbreaking little book called What Is Life? In fewer than one hundred pages, he argued that life was not a mysterious or inexplicable phenomenon, as many people believed, but a scientific process like any other, ultimately explainable by the laws of physics and chemistry.
Today, more than sixty years later, members of a new generation of scientists are attempting to create life from the ground up. Science has moved forward in leaps and bounds since Schrödinger's time, but our understanding of what does and does not constitute life has only grown more complex. An era that has already seen computer chip–implanted human brains, genetically engineered organisms, genetically modified foods, cloned mammals, and brain-dead humans kept "alive" by machines is one that demands fresh thinking about the concept of life.
While a segment of our national debate remains stubbornly mired in moral quandaries over abortion, euthanasia, and other "right to life" issues, the science writer Ed Regis demonstrates how science can and does provide us with a detailed understanding of the nature of life. Written in a lively and accessible style, and synthesizing a wide range of contemporary research, What Is Life? is a brief and illuminating contribution to an age-old debate.
In 1944, the Nobel Prize–winning physicist Erwin Schrödinger published a groundbreaking little book called What Is Life? In fewer than one hundred pages, he argued that life was not a mysterious or inexplicable phenomenon, as many people believed, but a scientific process like any other, ultimately explainable by the laws of physics and chemistry.
Today, more than sixty years later, members of a new generation of scientists are attempting to create life from the ground up. Science has moved forward in leaps and bounds since Schrödinger's time, but our understanding of what does and does not constitute life has only grown more complex. An era that has already seen computer chip–implanted human brains, genetically engineered organisms, genetically modified foods, cloned mammals, and brain-dead humans kept "alive" by machines is one that demands fresh thinking about the concept of life.
While a segment of our national debate remains stubbornly mired in moral quandaries over abortion, euthanasia, and other "right to life" issues, the science writer Ed Regis demonstrates how science can and does provide us with a detailed understanding of the nature of life. Written in a lively and accessible style, and synthesizing a wide range of contemporary research, What Is Life? is a brief and illuminating contribution to an age-old debate.
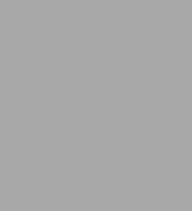
What Is Life?: Investigating the Nature of Life in the Age of Synthetic Biology
209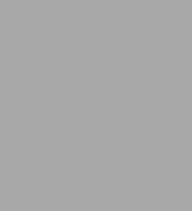
What Is Life?: Investigating the Nature of Life in the Age of Synthetic Biology
209eBookFirst Edition (First Edition)
Available on Compatible NOOK devices, the free NOOK App and in My Digital Library.
Related collections and offers
Overview
In 1944, the Nobel Prize–winning physicist Erwin Schrödinger published a groundbreaking little book called What Is Life? In fewer than one hundred pages, he argued that life was not a mysterious or inexplicable phenomenon, as many people believed, but a scientific process like any other, ultimately explainable by the laws of physics and chemistry.
Today, more than sixty years later, members of a new generation of scientists are attempting to create life from the ground up. Science has moved forward in leaps and bounds since Schrödinger's time, but our understanding of what does and does not constitute life has only grown more complex. An era that has already seen computer chip–implanted human brains, genetically engineered organisms, genetically modified foods, cloned mammals, and brain-dead humans kept "alive" by machines is one that demands fresh thinking about the concept of life.
While a segment of our national debate remains stubbornly mired in moral quandaries over abortion, euthanasia, and other "right to life" issues, the science writer Ed Regis demonstrates how science can and does provide us with a detailed understanding of the nature of life. Written in a lively and accessible style, and synthesizing a wide range of contemporary research, What Is Life? is a brief and illuminating contribution to an age-old debate.
Product Details
ISBN-13: | 9781429996280 |
---|---|
Publisher: | Farrar, Straus and Giroux |
Publication date: | 04/01/2008 |
Sold by: | Barnes & Noble |
Format: | eBook |
Pages: | 209 |
File size: | 421 KB |
About the Author
Ed Regis, who holds a PhD in philosophy from New York University, is a full-time science writer, contributing to Scientific American, Harper's Magazine, Wired, Discover, and The New York Times, among other periodicals. He is the author of several books, including The Biology of Doom.
Read an Excerpt
What Is Life?
Investigating the Nature of Life in the Age of Synthetic Biology
By Edward Regis
Farrar, Straus and Giroux
Copyright © 2008 Ed RegisAll rights reserved.
ISBN: 978-1-4299-9628-0
CHAPTER 1
Birth of a Cell
MAY 2005. In a new industrial park at Porto Marghera, some four miles across the lagoon from Venice, an American physicist by the name of Norman Packard is staring at the enormous 30-inch-wide display screen of a Macintosh G5 computer. Floating around against a dark background is a dense assortment of red, green, and blue dots.
"Blue is water, the greens are hydrophobic molecules, which means they don't like water, and the reds are hydrophilic molecules, which do," Packard says.
The simulation begins with the dots spread out evenly across the screen in a relatively homogeneous mix. But then in the incremental time-steps of the particle dynamics program, a pattern emerges. The greens move toward one another and then converge and clump together, forming a spherical structure. The reds, meanwhile, follow the greens and arrange themselves on the outside of the mass, as if to protect it from intrusion. The result is a vesicle, a tiny bilayered fluid-filled sac. The vesicle has formed itself spontaneously, the result of a self-assembly process driven by Brownian motion (the random thermal movement of molecules in a fluid medium) and by various chemical reactions.
"We believe that this combination of chemical reactions and self-assembly is one of the crucial combinations that we need to understand to make these artificial cells," Packard says.
Artificial cells? Venice? A city of more than a hundred churches, miles of canals, and innumerable ancient palazzi, all of them suspended in time, a place where nothing fundamentally new has happened for hundreds of years? Somehow the location is strangely fitting. In its heyday, Venice was a world-class power and trading center as well as a realm of considerable intellectual freedom. The city was now and always had been home to a variety of creative spirits: composers, artists, and scientists, including Galileo. And its labyrinthine streets and alleys were bathed in the green waters of the Venetian lagoon — water just coincidentally being the medium in which, according to most theories, earthly life originally began. So why should it not begin again, here?
Norman Packard, for one, finds no incongruity in the prospect. Packard is the chairman, CEO, and scientific head of ProtoLife s.r.l., a Venetian start-up company located in Parco Vega, a technology park the regional government had created on the grounds of an old chemical factory.
"The city of Venice, but even more generally the region of Veneto, wants to diversify its portfolio of activities," Packard said. "Venice has this very strong component of tourism that dominates its economy in many ways, and so it's trying to create some economic diversity that can give a certain kind of life to the city, not related to tourism."
ProtoLife's business plan is founded on an attempt to start life over, to begin from the beginning. It's not their intention to redo Genesis, outdo Frankenstein, or to blaze a path of glory through one of the final frontiers of applied science — although, if they're successful, Packard and his crew will end up doing all those things. The company's motivation is far more prosaic, practical, and commercial: to create artificial cells. Made from scratch and called "protocells," they will be programmed to carry out useful tasks such as synthesizing vaccines and drugs, cleaning up toxic waste, scavenging excess CO from the atmosphere, and other such miracles, and earning the company a tidy profit in the process.
After watching his simulation run a few more times — "We've done between six and seven thousand runs so far," he says — Packard walks down a polished green marble hallway, turns right, unlocks a door, and enters the company's lab suite. This is the domain of ProtoLife's chief chemist, Martin Hanczyc, a postdoc Packard recently hired away from Jack Szostak's competing artificial cell project at Harvard. In fact, ProtoLife is only one of a half dozen or so scientific efforts bent on creating new life: in addition to the ProtoLife and Harvard projects, there are others at Rockefeller University in New York, the University of Nottingham in England, and the University of Osaka in Japan, among other places. All too obviously, creating life is an undertaking whose time has come.
Hanczyc's laboratory at ProtoLife boasts a full supply of chemical apparatus: the usual lab glassware, serological pipettes, fume hoods, scales, centrifuges, microscopes, plus heavier machinery. "This is one of our main analytic tools, a combination spectrophotometer and fluorometer," Packard says of a large piece of equipment. "You find this in practically every chemistry lab in Europe, so we have one too."
Hanczyc has been synthesizing and studying various types of vesicles, and today Packard wants to show me what they look like. Packard is a big man with shaggy blond hair, glasses, and a courtly manner. He has a slow and deliberate style of speech, which includes a precise, mellifluous Italian, courtesy of his wife, Grazia Peduzzi, who was born in Milan. He squints through a fluorescence microscope, adjusts the focus, and finally, there they are: the real-life correlates of the objects he had been simulating on the computer.
"Somewhat dried up," he says of the vesicles, which Hanczyc had prepared a while ago.
A vesicle is not a living thing. It's just a shell, a husk, the merest framework of the full artificial cell that's supposed to assemble itself on the premises and spring into life at some undefined point in the future. Nevertheless, what we have here on the microscope stage is something passably astonishing, slight and rudimentary though it might appear at first glance. For these filmy minute blobs are the first stirrings of an event that last took place billions of years ago: the genesis of life.
THE DREAM OF creating life has ancient roots in the human imagination. In Frankenstein, which Mary Shelley completed in 1817 at the age of nineteen, the scientist Victor Frankenstein cobbled together a creature from body parts he'd spirited away in the dead of night from graveyards, dissection rooms, and slaughterhouses. The resulting beast came to life when Dr. Frankenstein, by unspecified means, infused "a spark of being into the lifeless thing that lay at my feet."
Serious scientific attempts at infusing a "spark of life" into inanimate flesh go back at least to Luigi Galvani's discovery in 1771 that by applying electrical currents to a dissected frog's legs he could cause them to twitch as if alive. A hundred years later, in 1871, Darwin spoke of life as possibly having arisen "in some warm little pond, with all sorts of ammonia and phosphoric salts, lights, heat, electricity, &c., present."
As if following Darwin's recipe, when twentieth-century scientists approached the problem of understanding how life originally arose on earth, they attempted to re-create what they thought were the original prebiotic conditions. The canonical effort, now a clichÃ(c) of twentieth-century science history, was the 1952 "Urey/Miller experiment," in which the chemists Harold Urey and Stanley Miller put ammonia, hydrogen, and methane inside a closed flask, circulated steam through this "atmosphere," and added bolts of "lightning" in the form of periodic electrical sparks. All they got for their trouble were some amino acids (building blocks of proteins) that were not in the mixture to begin with. The Urey/Miller experiment was once considered a very big deal, but it isn't by some of the protocell project's scientists: "We are not searching in the black and hoping that something happens," says the protocell researcher Uwe Tangen. "We're really trying to engineer these things."
Attempting to build an artificial cell is hardly a new idea in biology, but the specific protocell design Packard and Hanczyc are working on originated with Packard's longtime friend, the Los Alamos physicist Steen Rasmussen. Even as a boy in Denmark, Rasmussen liked to grapple with the big questions. He was by nature of a metaphysical turn of mind, and while still a kid he discussed subjects of cosmic import with his father, who was a bricklayer. Did the universe have a beginning — or an end? Where did it come from? Where was it going?
Later, in the 1980s, Rasmussen, together with Chris Langton, Norman Packard, and some others, became one of the founding fathers of the artificial life (ALife) movement. Launched at a Los Alamos workshop in 1987, artificial life was an attempt first to simulate and then actually to create a new life-form. Supposedly there was to be "soft," "wet," and "hard" artificial life, existing in the form of software, wet chemistry, and robotics, but the reality of the situation turned out to be quite different. "Most of the activities in the artificial life community have been with simulations," Rasmussen admits.
For a long time, that was true even of Rasmussen himself, who over the years had run countless computer simulations of various life- forms, modeling their possible self-assembly routes, evolutionary development pathways, and so on. But his abiding passion had always been to understand what life was and how it arose. At length he decided that the best way to understand life was to make some of it himself, ab initio.
In truth, he became obsessed with the idea. Although he lived in an adobe-style house surrounded by a number of natural life-forms, including his wife, Jenny, and three kids — not to mention horses, chickens, a parakeet, dog, and cat — he did most of his thinking at the Los Alamos lab and on his daily commute to and from, a route that took him past some of the most inhospitable, sun-blasted terrain imaginable: desiccated red cliffs, dry desert sands, and, occasionally, the whited bones of dead animals.
So daunting was the goal of creating new life, he realized, that only the simplest and most radically stripped-down design would have the remotest chance of actually working. Any given entity, in Rasmussen's view, had to have three main attributes in order to be considered alive: it had to take in nutrients and turn them into energy, meaning it had to have a metabolism; it had to reproduce itself; and its descendants had to be able to evolve by means of natural selection. A conventional biological cell, which did all that and more, was a masterpiece of complexity: it had an outer wall through which various essential substances were selectively transported in and out. It had an inner wall around the nucleus, which did the same. And both the nucleus and the cytoplasm surrounding it were brimming with all sorts of enzymes and other biochemicals, plus microstructures and organelles: the ribosomes, the mitochondria, the Golgi bodies, and all the rest. So very complex were even the simplest biological cells that it was a wonder they worked at all.
Rasmussen didn't want to get bogged down with all that incredible complication and detail, so he set about devising "the most lousy, simple, self-replicating, autonomous unit you can imagine," a cell so small it would be "the size of dust."
He got rid of the DNA, the nucleus, the organelles, and much of the rest of standard cell wetware. His protocell would be a minimal living entity, thousands of times smaller than a biological cell, and would be composed of three main structures: a container made of fatty acid molecules; a primitive metabolic system; and a new type of genetic material called PNA.
Fatty acids were also known as lipids, and their major attraction for Rasmussen was that "they make the containers for free. You put them in water and they make the containers. That's the state they want to be in. They want to join up and make these structures." The component molecules did this on account of their chemical polarity: one end of the molecule was hydrophobic (or water-avoiding), the other hydrophilic (or water-seeking), and so when placed in water the molecules naturally arranged themselves into little spongelike vesicles with the hydrophilic ends forming the outside surfaces and with the hydrophobic ends huddled together on the inside. (Many of the protocell's activities would be governed by the twin forces of hydrophobia and hydrophilia.)
For genes, Rasmussen needed a molecule that could both contain hereditary information in the manner of DNA or RNA, and could replicate, but without having to go through all the biochemical, biomechanical, and other enzymedriven contortions those molecules underwent in natural cells. What he needed, in short, was a coding molecule that could unzip and replicate in some quick and dirty, no-sweat, E-Z fashion. For this he chose PNA, peptide nucleic acid, a substance synthesized in 1991 by Peter Nielsen, the Danish biochemist. This was a double-stranded molecule that could split down the middle, just like DNA, uncovering its A, T, C, and G bases. Its advantage for Rasmussen, however, was the different ways in which the double-stranded and single-stranded versions of PNA behaved in the cell. A double-stranded stretch of it was hydrophobic and would sink down into the interior of the container and away from the water that surrounded the cell. At a preset temperature, the PNA molecule would spontaneously separate lengthwise inside the cell. The bases of the two single strands were hydrophilic and would therefore rise up to the cell's outer surface. There they would encounter matching PNA fragments that were also floating in the surrounding water, placed there with malice aforethought by the experimentalists. Those fragments would now attach themselves to the single strands, thereby forming new double-stranded molecules which, hydrophobic once again, would sink back down into the cell's innards. That took care of gene replication.
The protocell's metabolic, growth, and self-reproduction processes would be a product of light-sensitive lipid molecules being force-fed into the container. Light would activate the polarity of the molecules in such a way that their hydrophilic ends would rise to the container's surface and squeeze themselves in and among the other molecules that made up the cell's exterior. When the quantity of those surface molecules reached a certain critical mass, the forces holding them together would be overcome and the cell would split in half, reproducing itself.
Natural selection would come into the picture as the protocells reproduced: those that possessed some selective advantage in the speed or efficiency of replication would displace and ultimately wipe out those deficient in those qualities.
That was the basic design plan and operating formula of Steen Rasmussen's protocell. An ingenious design by any standard, especially if it worked. But in order to put his plan into effect, the wee matter of funding had to be addressed.
"I am doing this because I want to understand what life is," Rasmussen said. "That's the driver. Now that's not enough to get money, so the secondary driver is of course, Well, how can this be useful?"
From a practical point of view, there were three key benefits to Rasmussen's protocells. One was their relative safety: because artificial cells would be structurally and chemically alien to modern biology, they would be far less risky to experiment with than genetically engineered biological cells. As strictly nonbiological entities, "they'd have a much harder time interacting with modern life," Rasmussen said. "They'd be much less of an environmental or health hazard."
Second was their controllability: since they were designed to be programmable, the scientists ought to be able to coax the protocells to perform a larger range of tasks than was possible using ordinary cells and conventional biological engineering techniques. Suitably programmed protocells could unpollute the environment. They could act as "living pharmaceuticals," adapting themselves to a given individual's changing medical needs. They could produce new fuels, chemicals, structures, materials, and technologies.
Finally, because of the commercial value of those activities, they might even — unlike most other research projects financed by the government — make a profit.
AS IT TURNED OUT, money for such a far-fetched project was relatively easy to come by in Europe — provided that you and your organization were based there. Plus, office space for part of the effort was available for free in Venice, as Norman Packard learned while winding up his previous career in Santa Fe.
Norman was at this stage well into what might be called his Third Major Career Cycle (there were also smaller epicycles). Packard, who happened to be a cousin of David Packard, cofounder of Hewlett-Packard, had started out as a fairly conventional physicist, winning his Ph.D. at the University of California, Santa Cruz, in the late 1970s, after which he pursued a course of research into the main problem areas of the day: chaos theory, self-organizing systems, artificial life. That was his First Career Cycle. (As an epicycle to which, he and his friend Doyne Farmer designed and built miniaturized computer systems that were able to predict, fairly reliably, where a roulette ball would land after a spin of the disk. Never averse to making money with physics, Packard, together with Farmer and Mark Bedau, all of whom had been friends since their undergrad days at Reed College, secreted these devices on themselves and brought them into the casinos of Nevada — until they were busted by the gaming authorities.)
(Continues...)
Excerpted from What Is Life? by Edward Regis. Copyright © 2008 Ed Regis. Excerpted by permission of Farrar, Straus and Giroux.
All rights reserved. No part of this excerpt may be reproduced or reprinted without permission in writing from the publisher.
Excerpts are provided by Dial-A-Book Inc. solely for the personal use of visitors to this web site.
Table of Contents
Contents
Table of Contents,Title Page,
Prologue - The Second Creation,
One - Birth of a Cell,
Two - Schrödinger,
Three - Unlocking the Three Secrets of Life,
Four - The Fiftieth-Anniversary Coronation and Dismissal,
Five - ATP and the Meaning of Life,
Six - Origins,
Seven - The Spandrels of San Marco,
Eight - The Twilight Zone,
Nine - The Synthetic Cell Turing Test,
Ten - What Is Life?,
Also by Ed Regis,
Notes,
Bibliography,
Acknowledgments,
Index,
Copyright Page,
What People are Saying About This
Ed Regis is always a careful researcher, always an independent thinker. In this subversive little book, he shows that the biggest of big questions is still worth asking-more urgently now than ever. --David Quammen
Elegant, simple, clear, beautifully written. Regis takes up where Erwin Schrödinger left off and tackles the ultimate mystery of biology. This book is a scrumptious gem. --Richard Preston, author of The Hot Zone
A comprehensive and elegant analysis of the physical basis of life: an up-to-date successor to Schrödinger's 1944 book. --Marvin Minsky, Toshiba Professor of Media Arts and Sciences, M.I.T., and author of The Emotion Machine
Reading Group Guide
Questions for Discussion
1. Chapter one, "Birth of a Cell," describes Norman Packard's realization that funding for far-fetched projects was easier to obtain in Europe than in the United States. How do cultural distinctions between Europe and the United States influence the ways in which research and development are supported? What rules should govern the intersection of commerce and science?
2. What similarities and differences did you observe when comparing John McCaskill's microfluidic chips and human cells that develop organically?
3. What revolutionary perspective was embedded in Schrödinger's proposal that genes were messages written in code? What makes the concept of a coded "language" as the basis for molecular biology, "even today … a radical, almost fantastic notion," as the author describes it in chapter two?
4. "Unlocking the Three Secrets of Life" reminds us of the common fallacies "that DNA is a protein, that Watson and Crick discovered it, and that they did so in 1952." What accounts for the lack of popular knowledge about Friedrich Miescher's research on leukocytes, and the discoveries of such twentieth-century biologists as Avery, MacLeod, and McCarty? Does contemporary society require its scholars to be media savvy? What is the best way to bridge popular and scientific communities?
5. Did Schrödinger's interest in mysticism refute or enhance his scientific claims? Does the ideal of free will mesh with scientific doctrines? How did you react to his assertion (expressed in the opening paragraphs of chapter four) that there is God, or the potential for God, in every conscious mind?
6. How did chapter five change your understanding of metabolism and the cyclical nature of nature itself? What did you discover about the notion of energy expressed in the Krebs cycle?
7. What did you discover in chapter six, "Origins," regarding the relationship between religious beliefs and scientific beliefs among early researchers? How did Pasteur's Catholicism influence his attitude toward matter's ability to organize itself? Were you surprised to see Darwin quoted as stating that life had been "breathed by the Creator into a few forms or into one"?
8. The analogy of "The Spandrels of San Marco" was used by Gould and Lewontin to assert that not everything is an adaptation. Discuss the scientific turning point that is illuminated by this architectural metaphor, in which the spandrels are not designed to serve a specific purpose but instead simply arise as a byproduct. How could this approach-looking beyond design or adaptation-resolve intriguing quirks and mysteries you have observed about the natural world?
9. What is your explanation of self-sacrificial altruism in animals (human and otherwise)? Would you characterize the people in your life as altruistic?
10. Chapter eight, "The Twilight Zone," addresses the issue of synthetic biology, in which an organism's natural DNA may be partially replaced by one or more DNA sequences produced in a laboratory. At what point (if any) does such experimentation become unethical? How will the power of private-sector humanitarian organizations, such as the Bill and Melinda Gates Foundation, affect these debates?
11. In response to Niels Bohr's wry statement that "prediction is very difficult, especially about the future," discuss what the future may hold for the realm of biomedicine, technology, and other innovative fields. In one hundred years, what breakthroughs will surpass artificial hearts and the Internet?
12. What is your answer to the question posed at the end of chapter nine, "The Synthetic Cell Turing Test, " regarding the creation of artificial chemical cells: "Are the creators, the scientists involved, 'playing God?'" In the realms of medicine, agriculture, and beyond, what does it mean to "play God," and what is the best way to weigh benefits versus philosophical and physical dangers?
13. How do you personally answer the book's title question? Ultimately, how should we define "life"? What can we learn about ourselves and about the world around us simply by exploring the question?