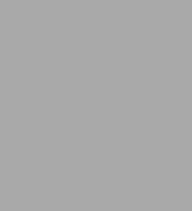
The Origins of Life: From the Birth of Life to the Origin of Language
192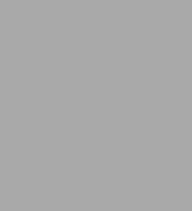
The Origins of Life: From the Birth of Life to the Origin of Language
192Paperback(New Edition)
-
PICK UP IN STORECheck Availability at Nearby Stores
Available within 2 business hours
Related collections and offers
Overview
The Origins of Life represents the thinking of two leading scientists on questions that engage us allhow life began and how it gradually evolved from tiny invisible cells into whales and trees and human beings.
Product Details
ISBN-13: | 9780192862099 |
---|---|
Publisher: | Oxford University Press |
Publication date: | 11/26/2000 |
Edition description: | New Edition |
Pages: | 192 |
Product dimensions: | 7.82(w) x 5.02(h) x 0.37(d) |
About the Author
Read an Excerpt
Chapter One
LIFE AND INFORMATION
Organisms are incredibly complex. The more we know about themtheir biochemistry, their anatomy, their behaviourthe more astonishing are the detailed adaptations that we discover. How could all this complexity have arisen? We can all understand that, if a farmer breeds from the cows that give most milk, the milk yield of the cows will in time increase. This depends on the fact that offspring resemble their parentsthat is, on hereditybut this does not at first sight present any great difficulty: we are familiar in our daily lives with the fact that children resemble their parents. Darwin's theory of evolution by natural selection amounts to no more than the idea that, in nature, those individuals best able to survive and reproduce will transmit the characteristics that enabled them to do so to their children, leading to the evolution of traits beneficial to the organism itself, rather than to the breeder. It is, perhaps, the one profound idea in science that we can all readily understand.
Although Darwin's idea is simpleperhaps because it is so simpleit is hard to believe that it can really explain the complexity we see around us. We may be able to breed cows that give more milk, but we could not breed pigs that fly, or horses that talk: there would be no promising variants that we could select and breed from. Where does the variation come from that has made possible the evolution of ever increasing complexity? Biology textbooks are liable to saythat mutationsthat is, new heritable variantsare random. The statement is near enough true, although 'random' is a notoriously difficult word to define: it would be better to say that, in general, new mutations are more likely to be harmful to survival than adaptive. Can it really be true that mutations that in their origin are non-adaptive led to the evolution of the wonderfully adapted organisms we see around us? This book is an attempt to answer that question. In trying to answer it, we shall be led to review a large part of modern biology.
The crucial step is to understand the mechanism of heredity, because the whole process of evolution by natural selection depends on it: if children did not resemble their parents, the Darwinian mechanism would not work. So, how can structures be replicated? The simplest way is by 'template reproduction'. If you wished to copy a metal statue, you would first make a clay mould of the statue and then pour molten metal into the mould, thus obtaining a copy of the original. This is an effective way of copying a surface, but would not work if the object to be copied resembled a living organism with a complex internal structure, down to the level of cells and molecules. In fact, when a complex organism reproduces, it first produces a simple egg, which then develops into an adult. The egg contains complex molecules, but no morphological structures similar to those of the adult: there is not a homunculus in the sperm.
How is it, then, that an egg develops into a mouse, or an elephant, or a fruit fly, according to the species that produced it? The short answer is that each egg contains, in its genes, a set of instructions for making the appropriate adult. Of course, the egg must be in a suitable environment, and there are structures in the egg needed to interpret the genetic instructions, but it is the information contained in the genes that specifies the adult form. The genes themselves can be copied by template reproduction: one cannot replicate an elephant directly by template reproduction, but one can replicate the instructions for making one and transmit those to the next generation. This is an idea that would have been strangeperhaps incomprehensibleto Darwin, but is less strange to us. We are familiar with the idea that patterns of magnetism on a magnetic tape can carry the instructions for producing a symphony, or that electromagnetic waves can be translated into an image on a television screen. (It is true that the TV image is two-, and not three-dimensional, but that is because of the difficulty of constructing a 3-D screen and not because of any difficulty in transmitting the information.)
The basic picture, then, is that the development of complex organisms depends on the existence of genetic information, which can be copied by template reproduction. Evolution depends on random changes in that genetic information, and the natural selection of those sets of instructions that specify the most successful organisms. But for this to work, the instructions must be interpreted. A compact disc needs a CD player, and the information carried by an electromagnetic wave needs a TV set to convert it into a moving image. Much of modern biology is concerned with how genetic information is translated into form: we describe some of the results below.
What is transmitted from generation to generation is not the adult structure, but a list of instructions for making that structure. As fish evolved into amphibians, or reptiles into birds and mammals, the instructions changed, essentially by random mutation and selection. But the medium in which the instructions were written, and the way in which they were translated into structure, remained basically the same. These processes were wholly unknown to Darwin: they are described below, particularly in Chapters 4 and 10.
But the crucial point we are making in this book is different. As life has become more complicated, the means whereby information is stored and transmitted have also changed: new coding methods have made possible more complex organisms. Thus the coding methods used by fish are not significantly different from those used by birds or mammals: only the message has changed, not the language in which it is written nor the means whereby it is translated. But if we view life on the largest scale, from the first replicating molecules, through simple cells, multicellular organisms, and up to human societies, the means of transmitting information have changed. It is these changes that we have called the 'major transitions': ultimately, they are what made the evolution of complexity possible.
This book is an account of the evolution of complexity. Although essentially Darwinian, it would seem very strange to Darwin, because it is couched in terms of genetic information and how it is stored, transmitted, and translated. This approach to evolution has led us to recognize several 'major transitions', starting with the origin of life and ending with the origin of human languagethe most recent change in the way in which information is transmitted between generations. Or perhaps it is not the most recent: we may today be living through yet another major transition, with unpredictable consequences.
What is life?
There are two ways to define life. The first is to say that something is alive if it has certain properties that we associate with living things on Earth; for example, if it grows or responds to stimuli. One problem with this approach is to decide which properties matter. If the first spacemen to land on Mars see an object walking towards them on six legs, with a front end bearing two lenses, a structure resembling a television dish, and a hole surrounded by sharp spikes, they will assume that it is alive, or perhaps an artefact made by creatures that are alive. But if they find that the rocks are covered by a purple slime, they will be less sure. A biologist would probably conclude that the slime was alive if it had a metabolismthat is, if the atoms that compose it were not a permanent part of its structure, but were being taken in from the surrounding environment, combined to form various chemical compounds, and later excreted back to the environment. All things on Earth that are commonly regarded as alive have this property of metabolism, whereas not all of them have legs, eyes, ears, and a mouth. So defining life in terms of metabolism seems sensible. But there are snags. Some things that are not alive have a metabolism of the kind just described, and some things that we might wish to think of as alive do not. We will return to these points later.
An alternative is to define as living any population of entities possessing those properties that are needed if the population is to evolve by natural selection. That is, entities are alive if they have the properties of multiplication, variation, and heredity (or are descended from such entities: a mule cannot multiply, but its parents did). The first two are easy to understand. An entity multiplies if it can produce two or more similar entities. Entities vary if, although similar, they are not identical. Heredity is a lot harder (Fig. 1.1). For the present, it is sufficient to say that entities have heredity if there are different kindsAs, Bs, Cs, and so onand if, when they multiply, As tend to give rise to As, Bs to Bs, and so on: that is, if like begets like. The occasional breakdown of heredity (mutation) gives rise to variation.
Why should we regard these three particular properties as defining life? It is because they are necessary if a population is to evolve all the other characteristics that we associate with life. Multiplication, variation, and heredity are not by themselves sufficient to guarantee the evolution of complex organisms. The environment must also be appropriate. For example, organisms able to walk would not evolve if there was no energy available to them, or if it was too hot to permit the existence of solid structures, or if there was too strong a gravitational field. In other words, evolution of particular structures depends on the environment, and, more generally, on the laws of physics and chemistry. But multiplication, variation, and heredity, even if they do not guarantee evolution, are at least necessary for it.
In this chapter, we explore further the relation between these two concepts of life, the metabolic and the genetic. In the last section we shall come back to a synthesis of these two, essentially complementary, views.
We said earlier that it is possible for an object to have metabolism, but not be alive. The object we had in mind was a fire. Atoms are continuously entering fires, in the fuel or oxygen supply, are involved in a series of chemical changes, and are leaving fires, mainly in carbon dioxide or water molecules. Yet while this is going on a fire may maintain a constant form, like the flame of a Bunsen burner with its blue centre and yellow margin. Fires also multiply. One can use a match to light a Bunsen burner, or even a Bunsen burner to set fire to a laboratory. They also vary in size, shape, and colour. Why, then, do we not think of fires as alive?
One possible answer would be that a fire must be supplied continuously with fuel or it will go out. Clearly this won't do: an animal also will die if it is not fed. One reason for thinking that a fire is not alive is that it is not sufficiently complicated and, in particular, because it does not have organs that ensure its survival and reproduction. Thus our spacemen identified the walking object as alive because it had partslegs, eyes, ears, mouthwith functions.
We would decide, then, that fire is not alive, but that the walking object on Mars probably is, because fire is too simple and does not have parts ensuring its survival and reproduction. What of the purple slime on the Martian rocks? We have already granted that the smear has metabolism, but so does fire. But if the smear is at all like living things on Earth, it has more than metabolism. Most of the steps occurring in living metabolism depend on enzymes. We will have more to say about enzymes later: for the present it is sufficient to say that they are large complex molecules that help to bring about specific chemical reactions, while themselves remaining unaltered. They are the chemical equivalents of the legs and eyes that persuaded us that the walking object was alive. They are organs that function to bring about the growth of the system as a whole. Specific enzymes could no more come into existence without natural selection than could legs or eyes.
We expect living things to have organs ensuring their growth, survival, and reproduction. We do not think of a fire as being alive because it lacks such organs. This is because fires lack heredity, and so do not evolve by natural selection. Fires vary, but the characteristics of a fire depend only on the supply of fuel and oxygen at the time, and not on whether the fire was lit by a match or a cigarette lighter. Lacking heredity, fire does not evolve, and so lacks the adaptive complexity that only natural selection can confer.
It is true that the object could also be an artefact, designed by intelligent beings. Interestingly, it is not in principle possible to tell the difference between a living organism and a product of intelligent design simply by looking at the object itself. If we are to distinguish between a living being and an artefact, it can only be by knowing its history. We could, if we wished, say that an object (a motorcar or a termite mound) is an artefact if it was constructed by entities (humans, termites) unlike itself, and a living organism if it is the product of evolution, although we would be in difficulty if someone constructed a living cell, indistinguishable from a real cell, in a test-tube.
It follows that the problem of the origin of life is the problem of how entities with multiplication, variation, and heredity could arise, the starting-point being the chemical environment of the primitive Earth. Given these three properties, the other characteristics we expect of living things will evolve. We discuss the origin of life Chapter 3. In this chapter, we make some general points about growth and heredity but, first, we must say a word about the possibility that life originated elsewhere in the Universe, either accidentally or by the deliberate action of intelligent extraterrestrials. This possibility cannot, we think, be ruled out. If it did happen, we are still left with the problem of explaining life's ultimate origin, which is not all that different from explaining its origin on Earth. In the absence of any evidence favouring an extraterrestrial origin, it seems sensible to treat the problem as a terrestrial one.
Autocatalysis
Before there can be heredity there must be reproduction, and before that there must be growth. The essence of growth is autocatalysis (Fig. 1.2). In an autocatalytic process, a chemical compound A undergoes a series of transformations, A [right arrow] B [right arrow] C [right arrow] D, etc. The crucial feature is that the last member of the series, say D, then produces two molecules of A. Thus each A molecule is the starting-point of a new cycle, leading to a new D, and so to two new As. Hence the concentrations of all the elements of the cycle increase. One can say that the chemical system is growing. In practice, a cycle of this kind will have side chains: a C will not always produce a D, but something else. Therefore the multiplication factor for each turn of the cycle will be less than two, but provided the flow into the side chains is not too great, the system will grow.
Autocatalytic cycles exist in nature: they are not just abstractions. Moreover, they are found outside living organismsa point that will become important when we come to discuss the origin of life. As early as 1861, the Russian chemist Alexander Butlerov described a reaction, the formose reaction, in which a solution of formaldehyde and sugars formed additional sugars at an accelerating rate. Both sugars and formaldehyde are simple organic compounds that are readily formed in the 'primitive soup' experiments described in Chapter 3. A second point is that any cycle of the kind shown in Fig. 1.2 requires an input of energy, in this case chemical energy. One cannot have a chemical perpetual motion machine any more than one can have a mechanical one. Again, however, this is not a problem on the primitive Earth. The action of solar radiation on the Earth's atmosphere would readily produce the fuel, including formaldehyde, needed to drive the cycle.
Autocatalytic cycles are important for the origin of life, because they would have produced a rich and varied chemical environment on the primitive Earth. But such cycles do not in themselves provide a mechanism of heredity. For heredity, it is not sufficient that an A should form two As. It is also necessary that, if an A should accidentally change to an [A.sub.1], say, then a new cycle, [A.sub.1] [right arrow] [B.sub.1] [right arrow] [C.sub.1] [right arrow] [D.sub.1] [right arrow] 2[A.sub.1], should result. If this did happen, then there would be two states, A and [A.sub.1], each capable of reproducing itself: this would indeed be heredity. But in general it will not be so. Instead, [A.sub.1] will be the start of a side chain, not producing more [A.sub.1], or even more A. The change will lead to degeneration, not to heredity.
It may occasionally be the case, however, that alternative autocatalytic cycles, A [right arrow] 2A and [A.sub.1] [right arrow] 2[A.sub.1], are possible, and that one could form the other by an accidental change, or 'mutation'. Since, almost inevitably, one cycle would be more efficient in utilizing the resources of the environment than the other, one would be 'naturally selected': there would be evolution by natural selection. But it would be a rather boring and limited kind of evolution, from A to [A.sub.1], or from [A.sub.1] to A. This brings up a crucial distinction between two kinds of heredity, which we call limited and unlimited heredity.
Limited and unlimited heredity
The case we have just described, of two competing autocatalytic cycles, is one of limited heredity. Although there are different kinds of entities, A and [A.sub.1], that can replicate, the number of possible kinds is not only finite but rather small. It is an advance over simple multiplication, in which a single type of entity increases in number, but it is not enough for evolution. Continuing evolution requires a system of unlimited heredity, in which an indefinitely large number of structures are each capable of replication. In all existing organisms, heredity depends on 'homologous base pairing' in nucleic acids, usually DNA. Leslie Orgel, a pioneer in the study of the origin of replication, once remarked that, as one traces life back to its origins, features disappear one by one, until one is left with homologous base pairing, like the smile on the face of the Cheshire Cat. The process is so important in the understanding of life that it is described in Fig. 1.3, although it will already be familiar to most readers. Base pairing is a mechanism of unlimited heredity. Given a million base pairs, there are 4 raised to the power one million possible structures that can be replicated, or many times the number of atoms in the Universe. It therefore provides an adequate basis for continued evolution.
A second distinction between kinds of hereditary mechanism is that between modular and holistic heredity. Heredity based on DNA is modular. A DNA molecule consists of many 'modules' (base pairs). If any one of these is changed, and the rest left unaltered, then the descendant molecules are changed just in that one module. In contrast, alternative autocatalytic cycles are an example of limited heredity, and they are holistic. There are no parts that can be changed without changing the whole replicating system. For example, in the case of an autocatalytic cycle, it is not just that A is changed to [A.sub.l], but all the intermediates are changed also, B to [B.sub.1], C to [C.sub.1], and so on. It is for this reason that a holistic system can replicate in relatively few states.
We believe it to be true that all systems of unlimited heredity will turn out to be modular. The statement is true not only of the genetic system based on DNA but also of the only other natural system of unlimited heredity known to us, human language. This is a system in which a small number of unit sounds (phonemes, roughly corresponding to the sounds indicated by the letters of the alphabet) can be strung together in different orders to express an indefinitely large number of different meanings. Changing a single letter changes the meaning of the whole: God's law and Sod's law are not the same, although sometimes one wonders. (We return to language in Chapter 13.) The claim that all systems of unlimited heredity are modular is also true of the many artificial ones, such as the Morse code and the ASCII code.
The mention of human language, or of the ASCII code, raises a query. We started by talking about the replication of structures and ended by referring to systems that transmit information. Much of this book is about the storage and transmission of information. What is the connection between the replication of structures and the transmission of information? Do all structures convey information? Does all information transmission depend on the replication of structures? The notion of information is so central to our purpose that it must be discussed in more detail.
Information and life
In the nineteenth century, society was transformed by machines that convert one form of energy into another. Steam engines convert chemical into mechanical energy, and electric motors and dynamos convert electrical into mechanical energy, and vice versa. These engineering inventions were accompanied by advances in theoretical science, thermodynamics, and electromagnetism. Sometimes the practical applications came first: the steam engine preceded an adequate theory of thermodynamics. Sometimes it was the other way round: Michael Faraday's elucidation of the relation between electricity and magnetism was the foundation of the electrical industry.
Society today is being transformed by machines that convert, not forms of energy, but forms of information. When you speak to a friend on the telephone, information in your mind is converted into a pattern of sound waves in the air, and then into a fluctuating electric current in the telephone wire. If the call is a transatlantic one, these fluctuations are then converted into electromagnetic waves (radio waves), before a series of reverse transformations occurs at the receiver, and your friend acquires the information that started in your mind. Despite the changes in material form from sound waves to fluctuating electric current, and then to radio waves, something is conserved throughout. That something is information. Not only telephones, but tape recorders, record players, radios, television sets, and computers are machines that translate information from one form to another.
These engineering developments have been accompanied by, and have to some extent been influenced by, the mathematical theory of information, although it is probably true that engineering applications have usually led the way. But perhaps the most important impact of information technology on pure science has been in biology, and especially in genetics. The influence is obvious from the terminology of genetics. Code, translation, transcription, message, editing, proof-reading, library, synonymous: these are all technical terms, with quite precise meanings in molecular genetics. The influence of information theory on biology has been in producing a way of looking at things, rather than in providing mathematical tools. One does not need to be a mathematician to work out that, if a code written in four kinds of bases is to specify 20 different amino acids, it must be at least a triplet code (a doublet code could specify only 4 x 4 = 16 amino acids). But the way of looking at things is crucial.
Should we think of a genethat is, a DNA moleculeas a structure that is replicated, or as information that is copied and translated? In present-day organisms, it is both: a gene plays two roles. It acts as a template in gene replication, so that two identical copies are made of a single model. If that were all, the DNA molecule would be just a structure that is replicated. But genes also act to specify the kinds of proteins a cell can make. This process is described in more detail in Chapter 4. For the present, it is sufficient that, via the genetic code, the sequence of bases in a gene determines the sequence of amino acids in a protein (the 'primary structure' of the protein); that this amino acid sequence determines, by the laws of chemistry, the way in which the protein folds up into a three-dimensional structure (the 'tertiary structure' of the protein); and, finally, that this three-dimensional structure determines what the protein does.
By an exact analogy with a tape recorder or a television set, we can say that the sequence of bases in a gene carries information that specifies the structure of a protein, just as the magnetic pattern on the tape specifies the sound that comes out of the recorder. Two points are worth making. First, the process is irreversible. There is nothing odd about this. Some information-transducing machines are reversible. One can use a tape recorder to convert sound into patterns of magnetism on a tape, or vice versa. Other machines are irreversibleyou cannot cut a record by shouting at a record player. The process whereby base sequence is converted into protein structure is irreversible. This is the explanation for the non-inheritance of acquired characters, a principle accepted by biologists for 50 years before a chemical explanation for it was available. We will revisit the problem in Chapter 10.
The second point is that translation requires translating machinery. A magnetic tape does not produce sound without a tape recorder, and a gene does not produce a protein without the translating machinery of the cell. We describe this machinery in Chapter 4. But where did it come from? The machinery consists of proteins (and also RNA molecules) whose structure is specified by genes. Unfortunately, this answer leads to an infinite regress: the translating machinery depends on the pre-existence of translating machinery, and so on ad infinitum. Philosophers do not like infinite regresses, but biologists are used to them, in the form of the classic chicken-and-egg problem. We discuss how the translating machinery may have originated in Chapter 4.
We have been describing how things are today. But, as we explain in Chapters 3 and 4, things were not always that way. The first replicating molecules, whether nucleic acids or something simpler, could not have specified anything, and so could not be said to carry information. They are best thought of simply as replicating structures. Only after the evolution of the translating machinery, and hence of specific proteins coded for by genes, is it sensible to talk of genes carrying information. Information theorists use the phrase 'information is data plus meaning'. In biology, the base sequence of nucleic acids provides the data, and the meaning is the structure and function of proteins.
The dual nature of life
We said earlier that there are two ways of defining life: the first is in terms of the complex structure of living organisms and, in particular, their possession of organs adapted to ensure survival and reproduction; the second is by the possession of those properties, especially heredity, needed for evolution by natural selection. We must now try to synthesize these two approaches. Aristotle asserted that life has a dual nature: material is provided by the egg and a formatting force (entelecheia) by the sperm. It is unfortunate that he expressed the idea in a manner that could serve as the basis for treating women as second-rate human beings (and, incidentally, in a manner that is in fact false, as far as the genetic contribution of the two sexes is concerned). But in emphasizing the two aspects of lifemetabolic and informationalhe was correct. As the American geneticist Hermann Muller remarked two millennia later, Aristotle's insight, although nor literally true, would have been worth a Nobel prize.
René Descartes argued that living beings were machines, and could be understood as such. This concept was characteristic of the seventeenth century. It is illustrated by one of the great triumphs of biology: the discovery by William Harvey of the mechanism of blood circulation driven by the heart. All biochemists and molecular biologists today are 'mechanical materialists'. But the machines they study differ from the ones imagined by Descartes. As the philosopher and mathematician Gottfried Leibniz was the first to point out, natural (or 'divine') machines are infinitely divisible. If we analyse a living organism, we find that it is composed of micro-machines (metabolic cycles, enzymes), whereas a steam engine has parts but is not composed of such micro-machines.
Another difference between artefacts and living machines noted by Leibniz is that there is always some entelecheia associated with the latter. The nature of this driving force was not further specified, but that it exerted some control on the system was clear to him. Much later, in his famous book What is life? (1944), the physicist Erwin Schrödinger emphasized this aspect of life. The book contains the famous remark that the gene, the information-carrying unit of the genetic material, must be an 'aperiodic crystal'. The genetic material must resemble a crystal in being stable and relatively inert, but it must also be 'aperiodic', in the sense of being composed of several different kinds of unit and not just of one kind of unit like a crystal of salt. The reason is that a string of identical unitsfor example, AAAAAcannot convey information, whereas a string of dissimilar units can. But Schrödinger also knew that living organisms must function. Today, we would express this aspect of life by saying that living systems cannot maintain their active state without a continuous influx of matter and energy.
The contemporary mathematical physicist Freeman Dyson, in a small volume entitled Origins of life (1985), revisited the problems posed by Schrödinger. He recognized that life required two things: a self-maintaining metabolic system and genetic material. He thought that concentrating on the latter had not given much insight into the origin of life, and advised that we should concentrate on the former instead. But what does self-maintenance mean? A living system is in continuous change, and some of the changes lead to degradation (that is why biochemists store their compounds in the freezer). It follows that, if the system is to maintain itself, it must be able to overproduce its own material. That is why the metabolic system must be autocatalytic: autocatalysis is needed for self-maintenance, let alone for growth and reproduction.
Some of these ideas were expressed earlier by Tibor Gánti, a Hungarian chemical engineer who became a theoretical biologist. As early as 1966, he argued that life consists of two sub-systems: a homeostatic-metabolic system and the 'main cycle', by which he meant informational control. In The principle of life, published in 1971, he described the 'chemoton', the basic design for a minimum chemical system showing all the characteristics of life. Oversimplifying, the chemoton consists of an autocatalytic chemical cycle, and an informational molecule (contained in a bag, so that constituents of the system could not float away in solution). According to this view, viruses are not alive. To use an analogy from computer science, viruses are like programs instructing computers to print them out in as many copies as possible, even if the computer is ruined in the process. It is not the virus, but the cell, that is analogous to the computer. A living being resembles a computer, rather than just a program, although it has its own program as a sub-system.
Gánti also discusses what are the criteria of life. By criteria, he means the empirically determined characteristics essential for life. One might object that some characteristic might be found in all living things on Earth, and yet be accidental. For example, it could have been the case that all organisms on Earth are light blue. How would you know whether blueness is necessary to life, or accidental? The empirical approach to the definition of life, therefore, runs the risk that some accidental trait will be accepted as essential. This should not worry us too much. All natural sciences have an empirical basis, and are therefore subject to modification when new data are discovered.
Gánti, then, adopted an empirical approach to identifying the defining criteria of life. He distinguished two types of criteria: 'absolute' and 'potential'. By an absolute criterion, he meant one necessarily present in all living creatures. A potential criterion is one that is not necessarily present in all living things, but that is necessary if organisms are to reproduce and evolve (the term 'potentiating', rather than 'potential', might express the distinction better). For example, mules are alive, but cannot reproduce. The ability to reproduce, therefore, is a potential but not an absolute criterion of life. Without going into the details of Gánti's argument, it is impressive that, very early, he recognized that both metabolism and informational control are necessary. Because we are interested in evolution, and not merely in the survival of individuals, we will concentrate on the potential criteriain particular, on multiplication and heredity.