Aquatic Pollution: An Introductory Text / Edition 3 available in Hardcover
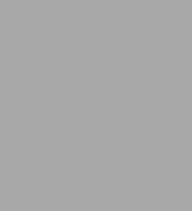
Aquatic Pollution: An Introductory Text / Edition 3
- ISBN-10:
- 0471348759
- ISBN-13:
- 9780471348757
- Pub. Date:
- 09/07/2000
- Publisher:
- Wiley
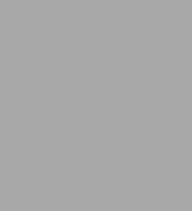
Aquatic Pollution: An Introductory Text / Edition 3
Hardcover
Buy New
$175.00Buy Used
$107.88-
-
SHIP THIS ITEM
Temporarily Out of Stock Online
Please check back later for updated availability.
-
Overview
A clear, straightforward presentation of concepts and issues in aquatic pollution
This comprehensive introductory text presents a systematic study of pollution in oceans, lakes, streams, and underground aquifers. In a clear, straightforward style that is easily accessible to nonscientists, it describes the sources, features, and effects of thirteen different types of aquatic pollution.
Fully updated to reflect current understanding and recent developments, this Third Edition of Aquatic Pollution covers every aspect of pollution associated with urban runoff, acid rain, sewage disposal, pesticides, oil spills, nutrient loading, and more. Case studies of major pollution sites such as Lake Erie, Three Mile Island, and the Rocky Mountain Arsenal help to illustrate points made in the general discussion. Important features of this new edition include:
* Updated discussions of nonpoint source pollution, industrial pollution, thermal pollution, pathogens, metals, plastics, and more
* New case studies of Chesapeake Bay and the Exxon Valdez
* Beginning-of-chapter outlines
* End-of-chapter study questions
* New special section on units of measurement
* Four chapters on the fundamentals of ecology and toxicology
Aquatic Pollution, Third Edition, is a first-rate teaching and learning tool for courses in environmental science, zoology, oceanography, biology, and civil or sanitary engineering. It is also an excellent primer for policymakers and activists focused on environmental issues.
Product Details
ISBN-13: | 9780471348757 |
---|---|
Publisher: | Wiley |
Publication date: | 09/07/2000 |
Edition description: | REV |
Pages: | 672 |
Product dimensions: | 7.05(w) x 10.28(h) x 1.43(d) |
About the Author
Read an Excerpt
Note: The Figures and/or Tables mentioned in this sample chapter do not appear on the Web.
Fundamental Concepts
- Rationale for the Study of Food Chains/Webs
- Food Chains
- Pathways of Organic Carbon
- Predator/Prey Relationships
- Use of Food
- biomass
- energy (respiration)
- Primary Production and Food Chains
- Low-energy Carbon --> High-energy Carbon
- Photosynthesis and Chemosynthesis
- Low-energy Carbon --> High-energy Carbon
- Autotrophs and Heterotrophs
- Primary and Secondary Production
- Grazing Food Chain
- Trophic Levels
- plants herbivores carnivores
- Ecological Efficiencies 20%
- Trophic Levels
- Ecological Pyramids
- Declining Biomass with Increasing Trophic Level
- Length of Food Chains
- Nonsteady State Inverted Pyramids
- Metabolic Rate and Size Effects
- Detritus Food Chain
- Excretion
- Detritivores
- Microbial Loop
- Balance Between Production and Consumption of Organic Matter
- Eutrophication
- P > R
- Cultural Eutrophication
- Food Chain Magnification (Biological Magnification)
- Food Webs
- Simple versus Complex Models
- Stability and Complexity
THE INTRODUCTION of pollutants into aquatic systems is a perturbation that can set off a complicated series of biological and chemical reactions. Some knowledge and appreciation of basic ecological concepts is necessary to understand and anticipate the nature of those reactions. Let's consider a simple example. Assume that an industry is discharging wastewater into an estuary. The wastewater contains mercury, which is a toxic metal. The mercury in the water reduces the photosynthetic rates of algae in the vicinity of the discharge.
Would the stress on the algae be the extent of the impact? Unfortunately, the answer is no. The reduction of photosynthetic rates would be only the first step. To the extent that photosynthetic rates were lowered, the food supply of herbivores would be reduced, and their biomass and production rates would also be lowered. Furthermore, the herbivores would assimilate some of the mercury absorbed by the algae and become stressed by the presence of the mercury in their tissues. Thus, the herbivores would be affected adversely both by a reduction in their food supply and by the presence of the mercury in their bodies. Using the same logic, it is easy to imagine how animals that preyed on the herbivores could be affected through similar mechanisms and how predator/prey interactions could ultimately spread the mercury to every organism in the water. Obviously, some understanding of the feeding relationships in a natural aquatic system is necessary to appreciate and anticipate the effects of such pollutants.
Now suppose that the mercury discharges ceased. Would the system recover and return to its original condition? Perhaps, but not necessarily. The stability of natural systems to perturbations such as pollutant discharges is a fundamental area of study in systems analysis and a critical consideration in the understanding of pollutant effects. The fact that a natural system is in equilibrium by no means guarantees that the system will return to its original state following a perturbation. To cite a popular example, had a very small meteor struck the Earth 65 million years ago, it is possible that a few dinosaurs might have been killed or injured. However, the dinosaur population probably would have returned to normal within a short time through natural processes. It is now generally agreed, however, that the extinction of all the dinosaurs was probably caused by a very large meteor that struck the Earth about 65 million years ago. Conditions on the Earth for a period of time following that event are believed to have been incompatible with the survival of dinosaurs, the result being that the system did not return to its pre-event status.
SIMPLE FOOD CHAIN THEORY
With this introduction, let's consider some basic ecological principles that relate to the movement and transformation of pollutants within aquatic systems. All animals require food. Food may be burned (respired) to provide energy or incorporated into the animal's body in the form of proteins, fats, carbohydrates, and other compounds to provide essential structural or metabolic components. Plants are by far the most important producers of food in most aquatic systems, although certain bacteria may be significant producers in some parts of the deep sea (Jannasch and Wirsen, 1977). Plants utilize sunlight as an energy source to manufacture organic compounds from carbon dioxide, water, and various inorganic nutrients in a process called photosynthesis. For example, a simplified equation describing the manufacture of glucose may be written
In this case glucose is the organic compound, the adjective organic meaning that the compound is found in organisms. If the reaction proceeds from left to right, the energy source is sunlight. Part of this energy is stored chemically in the glucose molecule. If the glucose is then oxidized by burning it with oxygen, the reaction proceeds from right to left and the energy stored in the glucose is released. Some of that energy is made available to the organism mediating the respiratory process and is used to perform various metabolic functions. It is common practice to use either organic carbon (C) or its associated chemical energy content as a metric for food supply, 1 gram of organic carbon being associated with an energy content of 8-11 kilocalories (kcal). All animals have the ability to transform organic compounds from one form to another and hence to convert their food into the compounds they require. However, only plants and certain bacteria have the ability to manufacture organic, high-energy compounds from inorganic, low-energy constituents, and it is this transformation that is referred to as primary production. If the energy needed to drive the transformation comes from light, the process is called photosynthesis. If the energy is obtained from chemical reactions involving inorganic compounds, the process is called chemosynthesis. Only certain types of bacteria and fungi are capable of mediating the latter process. All living organisms depend either directly or indirectly on primary producers as a source of food. Organisms that can produce most or all of the substances they need from inorganic compounds are called photoautotrophs or chemoautotrophs, depending on whether the energy needed to effect the conversion comes from light or the reactions of inorganic chemicals, respectively. Organisms that lack autotrophic capabilities are called heterotrophs. The production of biomass by heterotrophs involves the conversion of some form of organic matter (food) into living biomass and is called secondary production. Plants are autotrophs, and animals are heterotrophs. Most bacteria are heterotrophs, although some bacteria do have well-developed photoautotrophic or chemoautotrophic capabilities.
A plant-eating heterotroph, or herbivore, may consume food initially produced by a plant. The hervivore may in turn be eaten by another heterotroph, or primary carnivore, which converts part of the herbivore biomass into primary carnivore biomass. The primary carnivore may in turn be eaten by another heterotroph, or secondary carnivore, which in turn may be eaten by a tertiary carnivore, and so forth. Ecologists refer to such a system of successive food transfers as a food chain. Each component of the food chain is called a trophic level. In the example given, plants would make up the first trophic level, herbivores the second trophic level, primary carnivores the third trophic level, and so forth. Such a food chain is depicted schematically in Figure 1.1.
In most aquatic systems the transfer of food from one trophic level to the next is believed to occur with an efficiency of only about 20%. In other words, the rate at which food is ingested by a trophic level is about five times greater than the rate at which food is passed on to the next trophic level. This efficiency is referred to as an ecological efficiency, or more specifically as a trophic level intake efficiency (Odum, 1971, p. 76). Ecological efficiencies are generally low because much of the food ingested by a trophic level is either respired to provide energy or excreted because it cannot be incorporated into new trophic level biomass. However, ecological efficiencies are also reduced when, for example, an organism dies from disease or a female fish releases her eggs into the water. Eggs occupy a trophic level that is always lower than that of the organism that produced them.
Because ecological efficiencies are only about 20% in aquatic systems, the flux of food from one trophic level to the next steadily decreases as one moves up the food chain. The result is that the primary production rate is likely to greatly exceed the production of top-level carnivores, the magnitude of the discrepancy depending on the number of trophic levels in the food chain. Ryther (1969) has estimated that there are roughly six trophic levels in typical open ocean marine food chains. On the other hand, some coastal and upwelling areas may have food chains with as few as three trophic levels. This difference stems in part from the fact that the primary producers in open ocean systems are dominated by very small microscopic plants called phytoplankton, whereas in coastal and upwelling areas the individual phytoplankton cells tend to be larger, and the cells tend to form chains and gelatinous masses. In the coastal and upwelling areas the primary producers can therefore be efficiently grazed by rather large herbivorous crustaceans such as copepods or even small fish. However, in the open ocean, most of the phytoplankton are much too small to be consumed by crustaceans and small fish, and several intermediate trophic levels therefore separate these two categories of organisms. Regardless of the length of the food chain, the steady decrease in the flux of food to higher and higher trophic levels usually results in a decrease in the biomass of organisms on successively higher trophic levels. Thus, if one were to represent the biomass of each trophic level by a bar whose length was proportional to the biomass of organisms in the trophic level and if one were to lay these bars on top of each other, the resulting figure would look qualitatively like Figure 1.2. Arranged in this way, the bars of trophic level biomass form a pyramid, often referred to as an ecological pyramid.
There are two caveats relative to the issue of ecological pyramids. First, although Figure 1.2 correctly depicts the average distribution of biomasses in a food chain, it is quite possible in non-steady-state systems for the biomass distribution in two or more trophic levels to become temporarily inverted. In other words, trophic level biomass increases rather than decreases with increasing trophic level number. For example, in temperate oceans and lakes, a so-called bloom of plant biomass may occur in the spring as the water temperature and average daily solar insolation increase. This plant bloom generally does not occur at a time when the herbivore biomass is large, but the herbivore biomass begins to increase rapidly shortly thereafter in direct response to the increase in herbivore food. Typically, herbivore grazing reduces the plant biomass to a low level. Herbivore biomass peaks and then declines. The fall in herbivore biomass is caused both by the decrease in herbivore food and by grazing pressure from primary carnivores. Figure 1.3 shows qualitatively how plant and herbivore biomass may vary with time during this period.
A system in which the herbivore biomass is greater than the plant biomass for a short period following the plant bloom is shown in Figure 1.3. Such a condition may exist for a short time in many aquatic systems that are subject to large-scale seasonal cycles. During this period, the first two trophic level biomasses form a so-called inverted pyramid because the second trophic level biomass is greater than that of the first. This situation lasts for only a short time, and the average distribution of biomass is similar to that shown in Figure 1.2. The logical arguments that lead us to expect a normal pyramid of biomass do not necessarily apply in a non-steady-state system because, over short time intervals, predators may consume more food than prey are producing and hence reduce the prey biomass to a low level. Obviously, this situation cannot persist for long; otherwise, the predators would destroy their food supply. Hence, on the average, one does expect to see a normal pyramid of biomass.
A second point about ecological pyramids concerns the size of organisms making up each trophic level. In general, one expects predators to be larger than prey, and hence higher trophic level organisms should be larger than lower trophic level organisms. This expectation is generally fulfilled, although there are certainly exceptions to the rule (Longhurst, 1991). For example, animals that hunt in groups or packs, such as wolves or killer whales, may kill organisms larger than themselves. However, predators are usually larger than their prey, and as a result, the number of organisms on successively higher trophic levels decreases even more rapidly than the total biomass. Although it is generally true that large organisms consume more food than small organisms, it is also generally true that large organisms consume less food per unit biomass than do small organisms. The relationship between organism size and metabolic rate is such that, if two organisms differ in weight by a factor of 10,000, the larger organism can be expected to consume only 10% as much food per unit body weight as the smaller organism. In other words, the larger organism would consume about 1000 times as much food as the smaller organism, or 1,000/10,000 = 1/10 as much food per unit body weight.
Now consider a case in which the size of individual organisms on two successive trophic levels differs by a factor of 10,000, and the ecological transfer efficiency between the trophic levels is 20%. In this case, a steady-state situation might exist in which the total biomass of the second trophic level is twice that of the first. Although the second trophic level receives only 20% as much food as the first trophic level, the second trophic level needs only 10% as much food to support a given amount of biomass as the first trophic level. Thus, the logical arguments that lead us to expect an ecological pyramid of biomass need not apply to food chains in which the size of organisms on successive trophic levels differs greatly because these arguments implicitly assume the food requirements per unit biomass of all trophic levels to be identical. The fact that normal ecological pyramids of biomass are found in most natural aquatic food chains (e. g., Odum, 1971, p. 80; Sheldon et al., 1972) indicates that differences in organism size on successive trophic levels are not sufficiently great to invert the pyramids. Nevertheless, the difference in successive trophic level biomasses is often less than the factor of 5 that would be expected to result from transfer efficiencies of 20% if all organisms required the same amount of food per unit biomass (see question 1.8). Thus, organism size differences tend to reduce, but not eliminate, the effect of low ecological transfer efficiencies on trophic level biomass structure.
Recycling and the Microbial Loop
The food chain we have discussed up to this point is called the grazing food chain because the second and higher trophic levels consist of predators that graze upon prey. Primary producers occupy the first trophic level of the grazing food chain. A very important companion of the grazing food chain in any healthy aquatic system is the detritus food chain. The first trophic level in the detritus food chain is the nonliving organic matter produced by living organisms. This nonliving organic matter may exist either as particles or as dissolved organic substances and is referred to as detritus. The detritus provides food for a category of organisms called detritivores, a designation that includes both bacteria and certain metazoans. Bacteria have no mouthparts, and hence, strictly speaking, must feed entirely on dissolved organics. However, by exuding enzymes, they are able to solubilize and hence make use of particulate material as well. Metazoan detritivores such as benthic worms feed primarily on particulate detritus. Because detritivores are living organisms, they respire and excrete organics, just as do the members of the grazing food chain. The organic compounds excreted by detritivores may be utilized as food by other detritivores, and as a result only the most refractory organic compounds accumulate in the system. Most of the organic matter initially synthesized by the primary producers is ultimately respired, either by organisms in the grazing food chain or by detritivores. Animals or protozoans consume the detritivores, and in this way some of the organic C excreted by the grazing food chain is recycled back into the grazing food chain. The process is illustrated schematically in Figure 1.4. The portion of the detritus food chain involving dissolved organics, bacteria, and protozoans is often referred to as the microbial loop and is believed to account for much of the degradation of detritus in aquatic systems.
It is apparent from Figure 1.4 that the grazing food chain and the detritus food chain are interconnected and do not function independently of each other. The interaction between the two food chains is a mutualistic one, i. e., favorable to both and obligatory. The grazing food chain benefits the detritus food chain by excreting much of the organic matter needed by the detritivores for food; the detritus food chain benefits the grazing food chain by removing potentially toxic waste products excreted by both food chains. An approximate balance between the anabolism and catabolism of organic matter is essential to the maintenance of a stable aquatic ecosystem. In a system in which primary production on the average exceeds respiration, organic matter in the form of either plant or animal biomass or detritus will accumulate in the system. Eventually, the whole system may fill up with organic sediments. In fact exactly this process does occur, although often at a very slow rate, in most freshwater habitats and in some marine basins. This gradual accumulation of organic debris results in part from the fact that some detritus is rather refractory and is not broken down efficiently by detritivores. On the other hand, if respiration exceeds primary production, then a net consumption of biomass is occurring within the system. Such a system cannot persist unless it is subsidized by an external input of organic compounds, as, for example, from stream runoff.
It is important to realize that primary producers and detritivores utilize the waste products resulting from respiration and excretion, respectively, to create living biomass. For example, CO2, which is a direct product of respiration, is the source of carbon for primary production. Ammonia (as ammonium ions), which is excreted by many aquatic organisms, can be directly assimilated by primary producers as a source of nitrogen (N) for the production of proteins and nucleic acids. Waste products can be, and often are, toxic to the organisms that produce them. However, in a well-balanced ecosystem, waste products never reach high concentrations because they are constantly being utilized as a source of food by other organisms in the system. Detritivores play a crucial recycling role in aquatic systems by consuming organic wastes and converting them to inorganic forms that are utilized by primary producers. The grazing food chain utilizes the organic matter synthesized by the primary producers and releases part of it in the form of detritus, which in turn provides the food for the detritus food chain.
Due to this internal recycling, there is a tendency for both organic and inorganic compounds to accumulate in aquatic systems. Inorganic C can, of course, escape to the atmosphere as CO2, and inorganic nitrogen may similarly escape as ammonia or N2. However, under normal circumstances, the latter escape routes are not very efficient for N, and removal of organic compounds and essential nutrients via washout rarely occurs with 100% efficiency. The accumulation of refractory organic debris in the sediments and buildup of organic matter and nutrient concentrations in the water column are natural processes in most aquatic systems. Associated with these phenomena are increases in the rates of primary production and respiration and a decrease in the depth of the system caused by sediment accumulation. The whole process is referred to as eutrophication. Eutrophication eventually causes most lakes to fill up with sediments after a period of perhaps hundreds, thousands, or even tens of thousands of years. Sediments do accumulate at the bottom of the ocean, but they are removed by tectonic processes at subduction zones at rates that approximately balance their rate of formation. Obviously, there is no danger that the oceans will fill up with sediments. However, some regions of the ocean are much more productive than others, and this fact directly reflects the relative efficiency with which essential nutrients are recycled by the grazing and detritus food chain in different parts of the ocean.
Eutrophication is sometimes considered to be an unnatural phenomenon, but the imbalance between photosynthesis (P) and respiration (R) associated with eutrophication is nothing new. It was a fact of life on Earth literally billions of years ago. The atmosphere of the Earth was initially devoid of oxygen (O2), and the O2 in the atmosphere and ocean today is the product of photosynthesis. The first primitive plants evolved in the ocean, where the water shielded them from ultraviolet radiation. The O2 produced by those plants eventually accumulated in the ocean and atmosphere, and photochemical reactions in the atmosphere converted some of the O2 to ozone. The ozone in the atmosphere then became a shield against ultraviolet radiation. It was only after the establishment of this ozone shield that organisms were able to leave the ocean and evolve on land. Thus, the very habitability of the terrestrial environment today depends on the fact that there was an excess of photosynthesis over respiration on a grand scale during the early evolution of life on Earth. However, the imbalance between P and R has had other profound implications. O2 is one product of photosynthesis. The other product is organic matter. The imbalance between P and R during the geologic history of the Earth has resulted in the accumulation of both O2 and organic matter. Oil and coal deposits are obvious manifestations of the imbalance between P and R over geologic time.
Any unnatural acceleration of the eutrophication process due to the activities of humans is called cultural eutrophication. Cultural eutrophication can be caused, for example, by the discharging of sewage containing a high concentration of nutrients and organic matter. Instances of cultural eutrophication constitute one of the most common and widespread examples of water pollution problems. We will explore a few of these examples in detail in Chapter 4.
Food Chain Magnification
Respiration and excretion obviously play a critical role in controlling the flux of organic and inorganic materials between the grazing and detritus food chains. However, from the standpoint of water pollution, respiration and excretion are also important in determining the movement of pollutants both between and within these same food chains. If the pollutant is biodegradable, it may, of course, be catabolized and rendered harmless. However, if the pollutant is nonbiodegradable, it may be passed from prey to predator and in this way be spread throughout the grazing food chain. If some of the pollutant is excreted, then it may spread to the detritus food chain as well. One of the most important applications of food chain theory to water pollution problems has been the effort to explain how these transfers of a pollutant between food chains and trophic levels affect the concentration of the pollutant in organisms. In cases where it has been possible to examine in some detail the distribution of pollutant concentrations among the trophic levels in a simple food chain, results have sometimes indicated a steady increase in concentration with increasing trophic level number. Table 1.1 shows concentrations of the pesticide DDT (plus the closely related compounds DDD and DDE) in the water and in various organisms taken from a Long Island, New York, salt marsh. The residue concentrations increase steadily from the plankton to the small fish to the larger fish and finally to the fish-eating birds. The total concentration factor from plankton to fish-eating birds is roughly 600. Observations such as this one led some scientists to believe that a common mechanism or explanation might underlie similar observations of increasing pollutant concentrations at higher trophic levels in some food chains, a phenomenon that they termed food chain magnification.
LONG ISLAND SALT MARSH
a Parts per million (ppm) of total residues, DDT + DDD + DDE (all of which are toxic), on a wet weight, whole organism basis.
Source: Woodwell et al. (1967)
A logical explanation for food chain magnification is forthcoming from food chain theory if one assumes that certain pollutants ingested with an organism's food are not as effectively respired or excreted as is the remainder of the food. DDT would seem to be a likely candidate for such a pollutant because it is resistant to biological breakdown and tends to be stored in an organism's fatty tissues rather than being directly excreted with other waste materials that the organism is unable to utilize. Consider, for example, a case in which the ecological transfer efficiency of food between trophic levels is 20% but the transfer efficiency of DDT, caused by its resistance to respiration and excretion, is 60%. As a result, the steady-state concentration of DDT in a predator will be about three times greater than the DDT concentration in its food because the predator retains three times (60% versus 20%) as much of the DDT that it eats as it does of the remaining food. If this process is repeated through four trophic level transfers, the concentration of DDT in the fifth trophic level will be 34 = 81 times greater than the DDT concentration in the first trophic level.
Although this reasoning is logical enough, the logicality of the reasoning by no means guarantees that biological magnification of the sort described is responsible for observations such as those presented in Table 1.1. Pollutant concentration trends of exactly this sort may be produced by mechanisms very different from food chain magnification. Only carefully designed experiments can sort out the possible causes of such concentration trends. In Chapter 10 we will examine one such experiment in some detail. The point here is not to argue the pros and cons of the theory of food chain magnification, but rather to show how some knowledge about the characteristics of food chains can lead to a logical hypothesis regarding pollutant effects that is worth testing with further study. Logical thinking and hypothesis testing of this sort, based on sound ecological principles, represent the best means for studying and solving water pollution problems.
FOOD WEBS
Now that we have developed a simple food chain model as a conceptual basis for examining ecological problems, it is best to back up a step and remind ourselves that the world is not really so simple. The feeding behavior of many animals is such that they cannot be assigned to a unique trophic level. Some animals, such as shrimp, will eat almost anything they can swallow, including plants, detritus, and other animals. Obviously, they cannot be assigned to one or even a few trophic levels. Other organisms may feed on one trophic level as juveniles, on a second trophic level at a later developmental stage, and on a third trophic level as adults. In such a case, one would have to treat each developmental stage of the species as a different organism in order to make unique trophic level assignments. Certainly the kind and quantity of available food influence the feeding habits of many organisms. Figure 1.5 indicates the complex feeding relationships found in a small stream community.
The pattern of lines indicating the feeding relationships in such a system form a sort of web, and the feeding pattern has therefore come to be known as a food web. A food web depicts a more complex system than that represented by a food chain. One may therefore ask whether any of the implications deduced from food chain theory are relevant to a world that seems to be much more complex. The answer to this question is "yes" for the following reasons.
First, even though it is true that many organisms feed on more than one trophic level, it is also true that many organisms show a preference for one kind of food or another and can be reasonably defined as belonging to primarily one or two trophic levels. Although there are exceptions to this rule, the exceptions are not so numerous as to warrant discarding the concept.
Second, and perhaps more important, is the fact that some implications of food chain theory do not even require one to assign specific organisms to specific trophic levels. The idea that a large percentage of a prey will be either respired or excreted by the predator is valid regardless of the identity of the prey and predator. Thus, food in an abstract sense is produced by plants and is passed through a series of feeding transfers from one trophic level to the next, with roughly 80% of the food being respired or excreted at each transfer. Therefore, on the average, the biomass of food that has passed through m such transfers is likely to be much less than the biomass of food that has passed through m-1 transfers. If one thinks of trophic levels in terms of a series of food transfers, and not necessarily in terms of particular organisms eating particular organisms, the problem of assigning each organism to a unique trophic level disappears.
Despite this second point, it is in fact the case that the effect of pollutants on particular organisms is sometimes discussed with reference to an organism's position in the food chain. The relevance of food chain theory to such discussions is based on the first of the above two arguments, namely, that many organisms may be reasonably assigned to one or two trophic levels. For example, it is reasonable to assume that a pelican does not eat microscopic plants and animals, bacteria, protozoans, or even small fish; nor does a pelican eat whales, dolphins, or sea lions. Rather, a pelican eats fish of the size (approximately a few tens of centimeters) that can be conveniently scooped up in its mouth. Thus, a pelican would be assigned to roughly the fourth trophic level in a grazing food chain. Such reasoning, while not flawless, is unlikely to be wildly in error. Nevertheless, whenever food chain arguments are invoked, one should keep in mind that the theory has its shortcomings and that the feeding relationships of some organisms can be extremely complex.
FOOD WEBS AND ECOSYSTEM STABILITY
The complexity of aquatic food webs is associated with another controversy that is particularly relevant to the problem of water pollution. The controversy concerns the stability of ecosystems. To what extent will a biological community resist change when stressed by pollution, and will the system return to its original state if the pollution stress is removed?
In the 1950s and 1960s, a number of publications appeared in the literature suggesting that the complex interactions between organisms in a food web tended to stabilize the biological system. MacArthur (1955), for example, suggested that community stability might be roughly proportional to the logarithm of the number of links in the food web, a hypothesis that unfortunately has sometimes been accorded the status of a theorem. The hypothesis is based on conclusions derived from information theory, in which it is shown that such a logarithm provides a measure of the degree of organization or complexity. One then argues intuitively that the larger the number of links and pathways in the food web, the greater the ability of the system to damp down perturbations and hence the greater the chance that the system can absorb environmental shocks without falling apart. Examples of stable and very complex ecosystems include tropical rain forests and coral reefs.
A very elegant and understandable treatment of this issue has been provided by Robert May (1974). The somewhat counterintuitive result of May's analysis is that greater food web complexity per se does not impart greater stability to the ecosystem. In fact, the more complex the linkages in the food web, the more unstable the system is likely to become. As noted by May (1974, p. 75), "The greater the size and connectance of a web, the larger the number of characteristic modes of oscillation it possesses: since in general each mode is as likely to be unstable as to be stable (unless the increased complexity is of a highly special kind), the addition of more and more modes simply increases the chance for the total web to be unstable." The fact that tropical rain forests and coral reefs are highly complex systems may therefore be due more to the fact that these ecosystems exist in stable environments than to some inherent stabilizing characteristics conferred by the complex links in their food webs. May (1974) was careful not to rule out the latter possibility, and noted that some very special and mathematically atypical sorts of complexity might enhance ecosystem stability, but that it would be unwise to assume that, as a general rule, food web complexity implies stability. In fact, "If there is a generalization, it could be that stability [of the environment] permits complexity" (May 1974, p. 76).
An important point to bear in mind is that the foregoing discussion of stability and complexity pertains to complexity of food web linkages only. Other types of complexity may confer stability on ecosystems. For example, habitat complexity may provide refuges that allow some members of the biological community to survive adverse conditions. Similarly, complexity or diversity of the gene pool in a species may result in a subset of the population that is unusually resistant to a particular stress and hence better able to survive the impact of certain types of pollution. Similar considerations apply to the functionality of biological wastewater treatment systems. As we will see in Chapter 6, from a functional standpoint, a simple wastewater treatment system that relies almost entirely on bacteria to decompose the organic matter in sewage may be more easily perturbed by changes in the characteristics of the sewage than a system that relies on a more complex biological community. There are many types of complexity, and not all of them are inherently destabilizing. However, it appears from May's (1974) analysis that, as a general rule, increased complexity in food web linkages does not increase ecosystem stability.
Table of Contents
Fundamental Concepts.Photosynthesis.
Physical Factors Affecting Production.
Cultural Eutrophication--Case Studies.
Nonpoint Source Pollution.
Sewage Treatment.
Pathogens in Natural Waters.
Toxicology.
Industrial Pollution.
Pesticides.
Thermal Pollution and Power Plants.
Metals.
Oil Pollution.
Radioactivity.
Acid Deposition.
Groundwater Pollution.
Plastics in the Sea.
Glossary.
Units of Measurement and Abbreviations.
Index.