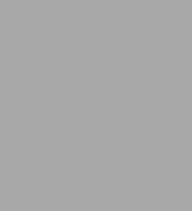
Bio-Synthetic Hybrid Materials and Bionanoparticles: A Biological Chemical Approach Towards Material Science
314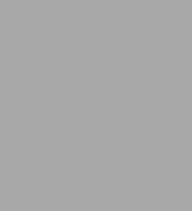
Bio-Synthetic Hybrid Materials and Bionanoparticles: A Biological Chemical Approach Towards Material Science
314Hardcover
-
SHIP THIS ITEMNot Eligible for Free ShippingPICK UP IN STORECheck Availability at Nearby Stores
Available within 2 business hours
Related collections and offers
Overview
Read an Excerpt
Bio-Synthetic Hybrid Materials and Bionanoparticles
A Biological Chemical Approach Towards Material Science
By Alexander Böker, Patrick van Rijn
The Royal Society of Chemistry
Copyright © 2015 The Royal Society of ChemistryAll rights reserved.
ISBN: 978-1-84973-822-4
CHAPTER 1
Synthetic Modifications of Proteins
ULRICH GLEBE, BARBARA SANTOS DE MIRANDA, PATRICK VAN RIJN, AND ALEXANDER BÖKER
1.1 Introduction: General Approaches to Protein Modification
Proteins are fascinating building blocks with outstanding functions in Nature. Bioinspired material synthesis utilizes these unique properties of proteins when integrated into synthetic structures. The properties of both the protein and the synthetic compound, inorganic or organic, can be combined to create novel hybrid materials for a broad range of applications. Proteins provide a multitude of applications, e.g. as catalysts, recognition sites, transporters, nanochannels and much more. Proteins are defined by their three-dimensional structures with distinct shape and size dimensions. Under suitable conditions, these structures self-regulate and can be re-formed into their natural shape. Unfortunately, this nearly perfect system in Nature is a disadvantage for the synthesis of biohybrid materials. Protein self-assembly is a sensitive feature and protein stability can decrease strongly under unsuitable conditions. To preserve the protein shape, including the resulting functions mentioned above, satisfactory conditions need to be found that allow the integration of synthetic components into protein-based systems in order to form biohybrid structures. In many cases, conditions that mimic the natural environment provide a suitable foundation. Generally, proteins are stable in aqueous media. However, not all chemical reactions needed for protein modification can be realized in pure aqueous systems. In some cases, organic cosolvents can be used to dissolve a wider range of chemical reactants. Nevertheless, the specific ratio for each organic solvent that conserves functionality and the overall folded structures of the protein requires attention. Additionally, heat, drastic changes in pH and certain chemicals cause denaturation of proteins.
The size, folding and function of proteins are predetermined by the precise sequence of amino acids. Several approaches are feasible to modify proteins selectively without influencing their functionality. The use of biotechnological methods allows for the exchange of specific amino acids or the deletion of some parts of the peptide sequence while retaining the protein shape and stability. Such changes can be used to add functional groups and also binding sites, to stabilize a protein or to alter the polarity of certain parts. Proteins can be integrated into organic or inorganic structures. By forming covalent or non-covalent bonds, small molecules, specific functional groups or even much larger nanoparticles can be attached to proteins. Particularly important are polymers, which are frequently used to modify proteins. The residues of amino acids which are oriented to the outside of the protein cage can be used for direct and covalent chemical modification. If polarity, charge and steric demand are not altered significantly, the protein remains comparatively stable with respect to its natural occurrence.
Hybrid materials offer the possibility to combine the beneficial properties of inorganic, bio- and polymeric structures to generate materials with novel functions. Applications and objectives for protein-based hybrid structures are drug/gene delivery, biosensing and bioimaging, in addition to creating materials for electronic devices and functional membranes. Furthermore, the conjugation to polymers may improve protein stability, solubility and biocompatibility and also increase the interfacial activity, decrease the amount of denaturation of the protein on an interface or render the proteins more compatible with other polymer structures. Over the past few years, bioconjugation strategies have been investigated intensively.
Protein–polymer systems are attractive for medical applications in the fields of diagnostics, therapeutics and theragnostics. Biocompatible or even biodegradable polymers could result in applicable hybrid systems. Although not yet ready for practical use, polymeric templates with enzymes and other proteins are envisaged to act as reaction spaces in nanoscale dimensions. Here, the insertion and encapsulation of biological compounds into polymer systems are possible, thereby protecting enzymatic reactions through the surrounding polymer structure. Such systems combine the stability and robustness of polymer structures with the specificity and selectivity that the biological compounds provide. Polymer conjugation can stabilize therapeutic proteins and protect them, especially from enzymatic degradation. It has recently been shown that a proline-specific endopeptidase (PEP) was stabilized to such an extent that it retained function even when presented with conditions as harsh as those found in the stomach. This was accomplished through the covalent attachment of a positively charged dendronized polymer.
For the conjugation of proteins to synthetic structures, complementary functional groups are needed. Two types of reactions are commonly used: polymerization and 'click' reactions. Polymers can be attached in one step (grafting-to approach) or the polymerization can be performed directly from initiator sites on the protein (grafting-from approach). Section 1.3 addresses the conjugation of polymers to proteins.
'Click' chemistry is a still fairly recent field that comprises bond-forming reactions with the aim of linking molecular building blocks via heteroatoms. In general, 'click' reactions are highly selective, easy to perform with a high tolerance towards the presence of other functional groups and solvents and lead to products that can easily be isolated in high yield with little or no by-product formation. Many 'click' reactions are biocompatible and can be performed in water, which makes them ideal tools for protein functionalization. The applicability to bionanoparticle modification was demonstrated shortly after the initial development of 'click' chemistry. The reaction between azides and alkynes to form a 1,2,3-triazole ring is one reaction of the 'click' chemistry pool, known as copper-catalysed azide–alkyne cycloaddition (CuAAC). Biological structures normally do not have alkyne and azide groups; however, they can easily be incorporated into proteins. CuAAC is a very suitable reaction for a specific ligation strategy for proteins and the most commonly applied 'click' reaction for bioconjugation [Scheme 1.1(a)]. However, the correct selection of the catalyst–ligand system, because of potential damage to biomolecular structures, and a comparably slow reaction rate at the low concentrations typically required for bioconjugation purposes must be emphasized. The copper-free variant of the reaction, strain-promoted azide–alkyne cycloaddition (SPAAC), provides a metal-free alternative for protein conjugation [Scheme 1.1(b)]. The activation barrier of the reaction is lowered by taking advantage of the inherent ring strain of cyclooctynes.
Copper(I)-catalysed 1,3-dipolar cycloaddition is the best known reaction, but other 'click' reactions are also commonly used and several of the reactions presented in this chapter for protein modifications indeed fulfil the requirements of 'click' reactions. Among them are the Diels–Alder reaction [Scheme 1.1(c)], Staudinger ligation [Scheme 1.1(d)], pyridyl disulfide, Michael addition and thiol–ene reactions [Scheme 1.1(e)], and also hydrazine and oxime formation. These copper-free 'click' reactions have the advantage that the toxicity and the possible denaturing character of CuI are avoided. Furthermore, Cu ions readily promote the generation of reactive oxygen species (ROS) that often harm biological structures. Sometimes proteins have to be functionalized first in order to apply a 'click' reaction. In other cases, native proteins can be used directly without modification, an example being the SH group of cysteine. The reaction of thiols with nonactivated alkenes can proceed via a radical (thiol–ene chemistry) or anionic (thiol Michael addition) pathway. Additionally, 'click' reactions are well suited for the surface immobilization of small molecular components, including fluorescent labels, and also polymers on protein structures.
1.2 Amino Acid Targeting for Synthetic Protein Modification
Since the functional groups of chemical compounds, including polymers, are highly diverse, the chemical strategy for the synthesis of protein hybrid conjugates is predetermined by the amino acid residues of the protein. First, 10 out of the 20 natural amino acids bear functional groups that are suitable for ligation chemistry. Second, it is important to consider whether or not these amino acids are accessible on the protein surface for chemical modifications. Lysine and cysteine are the two amino acids most commonly used for conjugation. In addition, tyrosine, glutamine, tryptophan, histidine, aspartic acid, glutamic acid, arginine and phenylalanine plus the N- and C-termini of the peptide chain are also possible reaction centres. For the other amino acids, almost no specific reaction that proceeds without damaging the native state of the protein or competing with functional groups of other amino acids is known. Furthermore, the modification of a specific amino acid residue should not influence the conformation and function of the protein.
Amino acids that are embedded in the globular structure of a protein are not accessible for chemical reactions. The average surface accessibility (ASA) indicates whether amino acids are addressable by chemical reactions. On average, aspartic acid, lysine, glycine, glutamic acid, glutamine and serine are mostly located on the surface of proteins. If one amino acid is accessible multiple times on the protein surface, this can lead to undefined and polydisperse products with varying degrees of modification. As a result, the monodisperse character that is a defining feature of proteins is lost.
The lysine residues and the N-terminus of proteins or peptides are the most popular sites for polymer conjugation. Lysines not only have a common location on the surface of proteins, but additionally can be used in a range of chemical reactions. The nucleophilicity of the amine group is higher than those of nucleophilic groups of other amino acids. Reactions with activated carboxylic acids are commonly used. For this purpose, N-hydroxysuccinimidyl (NHS) esters are widely utilized in addition to other carboxylic acid derivatives such as NHS carbonates, NHS carbamates, anhydrides and acid halides. In addition to such acylations, alkylation is also used for lysine modification. Here, a charge on the amine group can be retained, for example through amidation with imido esters. This may be advantageous if the charge on the residue is important for the stability and structure of the protein. Aldehydes react reversibly with lysine groups to form imines that can be reduced to amines under mild conditions. Scheme 1.2 gives an overview of common products of lysine modifications. In general, most proteins possess many accessible lysine residues. This, however, is accompanied with the loss of monodispersity due to differing degrees of amine modification, as mentioned earlier. For different proteins, the reactivity of lysine residues varies significantly owing to accessibility and pKa values. By adjusting the pH, one can influence whether the reaction takes place at the amine group of lysine (pKa ≈ 10.5) or at the N-terminus of the protein (pKa ≈7.8). However, in practice this is very difficult to achieve.
Cysteine is also often used for conjugation and usually does not have the disadvantage of forming polydisperse bioconjugates. Cysteine is present in only small amounts on the protein surfaces and there are especially few cysteine residues that do not participate in disulfide bonds. In combination with the possibility of using reactions that are not feasible with other amino acids, the small amount of surface amino acids can be exploited for selective modification. Two reaction pathways are common for cysteine modifications: the formation of disulfide bonds and alkylation during a Michael addition or thiol–ene coupling (Scheme 1.2). Activated disulfide groups such as orthopyridyl disulfides or methanethiosulfonates react with cysteine residues with the formation of disulfide bonds. Reducing agents can cleave the disulfide linkages formed, which can be an advantage or disadvantage depending on the desired application. Activated alkenes such as maleimides and vinyl sulfones form stable thioether bonds with thiol groups in Michael additions. Recently, it was shown that also mono- and dibromomaleimides and electron-deficient alkynes can be used for conjugation to cysteine residues. Sometimes disulfide bonds have to be cleaved to make them accessible for ligation. It is possible to reduce selectively the surface-accessible disulfide bridges only. In many cases, the removal of a disulfide bond in a protein influences the overall three-dimensional structure. However, possibilities exist for preserving linkages in these positions by linking the two sulfur atoms via a three-carbon bridge. After cleavage of the disulfide bridge, a reaction with a bis(thiol)-specific reagent stabilizes the correct tertiary structure of the protein.
In addition to the common protein modification approaches using lysine and cysteine residues, further chemical reactions addressing the residues of other amino acids should be mentioned that may be needed especially when the surface of the protein structures is devoid of any conventional addressable functionality. Tyrosine can be modified by palladium-catalysed alkylation of the hydroxyl group, by an electrophilic aromatic substitution such as a Mannich-type reaction or by a diazonium coupling. However, these approaches have rarely been used up to now. For glutamine, only enzymatic reactions mediated by transglutaminase are available for modification. The indole ring of tryptophan can be used for rhodium-catalysed reactions in which a rhodium carbene is formed in situ. Histidine residues form stable complexes with divalent transition metal ions. So-called His tags are composed of six histidine residues in a row and are used as a common binding motif for metal ions in peptide chemistry. In order to bind chemical compounds via this motif, these compounds need also to be equipped with a metal binding site. Carboxylic acid groups of aspartic acid and glutamic acid and the C-terminus of proteins are reacted with amines in reverse to lysine modification. This is achieved by enzymatic and biosynthetic approaches. The carboxylic acid group can be converted to very different chemical groups that may be suitable for further modification.
For a more detailed overview regarding the synthetic modification of amino acids in proteins, the reader is referred to reviews by Jung and Theato,24 Canalle et al. and Gauthier and Klok.
1.3 Synthetic Approaches for Polymer–Protein Hybrid Structures
The modification of proteins with polymers is one of the most widespread approaches to create hybrid structures and offers tremendous possibilities for functional systems. Both polymerization and coupling reactions are used to achieve polymer structures on protein surfaces. First, three major strategies have to be distinguished (Scheme 1.3). The grafting-to approach involves the synthesis of polymer chains with suitable end-groups that are then linked to proteins. Protein-reactive groups can be introduced to polymers in a post-polymerization step or protein-reactive initiators are used directly for the polymerization reaction. The latter guarantees that each polymer chain indeed has a protein-reactive end-group. The biomolecules are only involved in the last synthetic step as the polymers are synthesized separately. An excess of polymer is needed for the conjugation reaction, which hampers purification of the protein–polymer hybrid. Unreacted polymer and maybe even protein have to be removed from the conjugate. This disadvantage can be avoided with the grafting-from approach. Here, the polymer chains grow directly from a protein. First, small initiator molecules are linked to the protein surface able to initiate the polymerization from these so-called macroinitiators. Hence the purification of the product is easier because only small molecules, unreacted monomer and the catalyst, have to be removed. Furthermore, the location of the polymers is defined by the initiator positions and steric hindrance is negligible. Particularly in cases where it is intended to link a high density of polymer chains to a protein, grafting-from is superior to grafting-to because the steric hindrance of the growing, initially short, polymer chains is much smaller. Additionally, the synthesis of hydrophobic polymer–protein conjugates and the preparation of conjugates with high molecular weight homopolymers or block copolymers are easier via grafting-from. The drawback associated with the grafting-from approach is that polymerization conditions have to be chosen that do not affect the activity and folded structure of the protein. The third possibility is the grafting-through strategy. Here, monomers that contain proteins/peptides are polymerized so that every repeat unit of the comb-shaped polymer structure has a protein/peptide moiety. Grafting-through potentially allows the incorporation of a high amount of proteins/peptides into the conjugates. Alternatively, monomers with a protein/peptide binding site can be polymerized and the biocomponents attached to the polymer backbone in a post-polymerization step. More details and examples of the use of the different grafting strategies are given below.
(Continues...)
Excerpted from Bio-Synthetic Hybrid Materials and Bionanoparticles by Alexander Böker, Patrick van Rijn. Copyright © 2015 The Royal Society of Chemistry. Excerpted by permission of The Royal Society of Chemistry.
All rights reserved. No part of this excerpt may be reproduced or reprinted without permission in writing from the publisher.
Excerpts are provided by Dial-A-Book Inc. solely for the personal use of visitors to this web site.