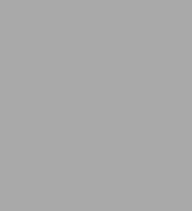
Green and Sustainable Medicinal Chemistry: Methods, Tools and Strategies for the 21st Century Pharmaceutical Industry
272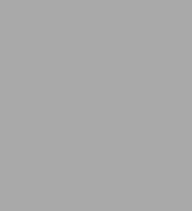
Green and Sustainable Medicinal Chemistry: Methods, Tools and Strategies for the 21st Century Pharmaceutical Industry
272Hardcover
-
SHIP THIS ITEMTemporarily Out of Stock Online
-
PICK UP IN STORECheck Availability at Nearby Stores
Available within 2 business hours
Related collections and offers
Overview
About the Author
Louise Summerton is the Training, Education and Networks Manager in the Green Chemistry Centre of Excellence (GGCE), University of York, UK, where she is leading on the creation of the CHEM21 Education and Training package to promote the uptake of green chemistry in the pharmaceutical industry.
Helen F Sneddon is a medicinal chemist as GlaxoSmithKline, UK, where she founded and leads the GSK Green Chemistry Performance Unit, which looks at improving the environmental sustainability of research and development, and the routes arising from it.
James H Clark is Professor of Chemistry at the University of York, Director of the Green Chemistry Centre of Excellence, and a Director of the Biorenewables Development Centre, UK. He has been at the forefront of green chemistry worldwide for nearly 20 years.
Read an Excerpt
Green and Sustainable Medicinal Chemistry
Methods, Tools and Strategies for the 21st Century Pharmaceutical Industry
By Louise Summerton, Helen F. Sneddon, Leonie C. Jones, James H. Clark
The Royal Society of Chemistry
Copyright © 2016 The Royal Society of ChemistryAll rights reserved.
ISBN: 978-1-78262-467-7
CHAPTER 1
Green and Sustainable Chemistry: An Introduction
JAMES H. CLARK
Green Chemistry Centre of Excellence, Department of Chemistry, University of York, York YO10 5DD, UK Email: james.clark@york.ac.uk
1.1 What is Green Chemistry?
"Green Chemistry", "Green Engineering" and "sustainability" are often used interchangeably to describe the concept of making processes and products that have less environmental impact and are (ideally) based on renewable resources. However, if one examines these concepts more deeply, it will become clear that there are significant differences in their philosophy, which impacts the applicability of the methodologies and techniques in the development of an environmentally appropriate society.
Green Chemistry is quite well defined by the twelve principles of Anastas and Warner. These principles focus mostly on how one should carry out chemical reactions and make chemical products, and describe the synthesis of chemicals in an environmentally preferable way. Thus, specific ideas, such as the use of benign auxiliaries including solvents for reactions and separations, reducing the number of steps, and the concept of atom economy, or incorporating all of your raw materials into your product, are focal points of Green Chemistry. The 12 Principles were written 20 years ago and don't fully reflect the modern way of thinking. Additional issues, such as toxicology and biodegradability, now play an important role in Green Chemistry as part of a greater emphasis on product safety as well as on renewable resources. In many respects, Green Chemistry can be considered as the scientific underpinning of environmentally preferable manufacturing.
Green Engineering, on the other hand is the design, commercialization and use of processes and products that are feasible and economical while minimizing generation of pollution at source, as well as minimizing risk to human health and the environment. The green engineer uses the tools of recycling, process intensification and design optimization to maximize the efficiency of a process and reduce its burdens on the environment. Green engineering evaluates the manufacturing process as a system and seeks to optimize its design, and in the truest sense, incorporates the concepts of lifecycle analysis and environmental economics into an appropriate evaluation of the overall environmental impact. Green engineering requires the development of a set of metrics that appropriately evaluate the environmental parameters that we seek to control.
Sustainable design looks even more broadly to try to understand the relationships between the manufacturing system and the ecosystem. Sustainability focuses on the triple bottom line: the integration of ecological integrity, societal responsibility, and economic viability. Sustainability takes the broadest level systems approach, looking at the planet as the system of interest, but in order to optimize design at this scale, new ways of measuring human impacts on the environment will be required.
The chemical and allied industries now face as tough a challenge as they have ever faced. The 20th century saw enormous growth in chemicals manufacturing but this growth has come at a cost. Inefficient processes leading to unacceptable levels of pollution, hazardous operations resulting in a number of disasters, and a lack of knowledge of the human and environmental toxicity of most chemicals in widespread use, all leading to an exponential growth in chemicals legislation. The industry now needs to achieve environmental and social acceptability as well as economically viable manufacturing in the toughest-ever legislative framework. Recent chemicals legislation, such as REACH, is causing major changes in the chemical product supply chain. Yet somehow this has to be done in a way that satisfies the demands of a growing population. Sustainable chemical production can only be realized through a reassessment of the entire chemical product lifecycle from resources, to manufacturing and production, through to product use and ultimate fate (Figure 1.1).
1.2 Drivers for Change
1.2.1 Legislation
Pressure on chemical manufacturing, especially legislative and also from customers, continues to be applied and generally leads to cleaner and safer manufacturing. The chances of another disaster such as Bhopal are lower, at least in most regions of the world (e.g. as a result of penalties and restrictions over the storage of hazardous substances) although there is still considerable manufacturing in regions with less control and therefore higher risk. The control over pollution and the severe penalties that can be imposed have discouraged significant emissions from plants in most locations.
REACH is the most talked about legislation affecting chemical products. This and other chemical legislation directly affect chemical and allied manufacturing through restricted availability of an increasing number of common chemicals. While very hazardous substances such as organomercury and lead compounds have been the subject of rigorous scrutiny for many years, new restrictions on the use of others such as chromates and cobalt compounds may have considerable impact on some industrial chemistries, including oxidations. While REACH is slow in its progression (it will be the next decade before all the chemicals subject to REACH have been tested), unofficial lists of substances to substitute have appeared. Probably the most prominent of these is the so-called SIN ("substitute it now") list. Several hundred chemicals appear on this list and it is influencing some end-users who do not want their products to contain any chemicals on such publically available "red lists".
Perhaps the biggest impact will be over the use of solvents since many of the more common organic solvents are under threat from REACH: these include N-methyl-2-pyrrolidone (NMP), dimethylformamide (DMF) and dimethylacetamide (DMAc). (For more information on solvent substitution guides see Chapter 2, 'Tools for Facilitating more Sustainable Medicinal Chemistry', by Helen Sneddon and James Sherwood's Chapter 3 on renewable solvent selection.) The electronics industry has also been subject to chemical legislation that aims to replace especially hazardous substances. RoHS (restriction on hazardous substances) targets certain chemicals, including lead, mercury, cadmium chromates and polybrominated flame retardants.
1.2.2 Elemental Sustainability
In addition to substances becoming restricted or unavailable due to changes in legislation, they may also be at risk due to issues with supply. Elements used by the chemicals industry, both in the manufacturing steps (e.g. as catalysts) and in the products themselves, include organohalogen compounds and numerous organic compounds containing heteroatoms, such as phosphorus, sulfur and boron. These are extracted from virgin ores and other natural sources, which, like petroleum, are finite and require a large amount of energy for extraction. While renewable carbon has been a hot topic for the last decade (see the next section), it is only in the last couple of years that attention has been broadened to include other critical elements including phosphorus and many metals (see Chapter 5 on elemental sustainability by Andrew Hunt). Some of the elements of concern are listed in Table 1.1. Many elements are now considered to be under threat in terms of predicted usage rates and known reserves.
Some may run out within 10 years (e.g. indium and germanium). While new reserves are being discovered, as with oil they are often of relatively poor quality and come at high economic and environmental costs. It is ironic that some shortages (e.g. lithium and some of the rare earths) are a result of increasing rates of use for low-carbon technologies. While we certainly need low fossil carbon technologies, we must introduce these with our eyes wide open to any consequential issues, such as high use of other critical elements.
One way that researchers are responding to the criticality of some elements is by much smarter use of these elements, i.e. better catalyst design and recycling (discussed in Chapter 11), and by developing catalysts that avoid the use of critical elements by focusing on the use of more plentiful base metals (Chapter 16).
1.2.3 Renewable Resources
Manufacturing of chemicals is resource-dependent. Petroleum has dominated the industry as a carbon feedstock with a few exceptions, including a small percentage of naturally derived compounds (e.g. for use in personal care products and pharmaceuticals) and chemicals derived from coal in South Africa (developed to overcome trade barriers introduced in the apartheid era). There is increasing pressure, especially from consumers, on manufacturers to produce bio-derived chemicals as replacements for fossil resources and substances now considered to be hazardous to us or to the environment. The move towards bio-based products is considered to have a number of advantages:10
Use of renewable and expendable resources
Less dependency on limited and increasingly expensive fossil resources
The potential to reduce greenhouse gas emissions (carbon neutral/low carbon impact)
The potential for sustainable industrial production
Potentially improved community health
Supports rural development
Increased industrial competitiveness through innovative eco-efficient products
Potential for transfer to other regions of the world, including the transfer of appropriate technologies discovered and proven in the EU
Vijayendran recently estimated that by 2025 over 15% of the $3 trillion global chemical market will be derived from bio-sources. Active pharmaceutical ingredients (APIs), polymers, cosmetics, lubricants and solvents have also been estimated to be the most important sub-segments of the chemical sector by the ad hoc advisory group for bio-based products. APIs in particular, with 33.7% of global chemical sales, are expected to be the chemical segment with the highest percentage sales of products produced using biotechnological processes. As we begin to move away from petrochemicals, the use of biomass as a chemical feedstock will become increasingly important.
1.3 Biomass as a Chemical Feedstock
Biomass is generally understood to mean large-volume, low-value bioresources that can be used as feedstocks for making chemicals, fuels and materials. To distinguish biomass from fossil resources, such as coal and petroleum (themselves ancient biomass), it is prudent to limit ourselves to resources that are less than 100–200 years old (resources that have a similar lifecycle to man). In this way, we can consider biomass to include:
Forestry residues
Short rotation trees
Agricultural residues including straws
Food processing wastes including shells, stones, peels
Grasses and other land grown biomass not used for food
Marine residues
Macroalgae (seaweed), microalgae and other water-grown biomass not used for food
Other food wastes
The total amount of this available biomass is not precisely known but has previously been estimated to be 50 billion tonnes per year, including 1.3 billion tonnes per year of food waste.
We can classify biomass into 3 main categories:
Carbohydrate (starch, cellulose and hemicellulose) including lignin from lignocellulosic biomass
Triglycerides (soybean, palm, rapeseed, sunflower oil)
Mixed organic residues
Lignocellulosic biomass consists of dry plant matter containing cellulose, hemicellulose and lignin. It can be sourced from a variety of dedicated crops, such as miscanthus, willow or poplar. Alternatively, feedstocks can be obtained from wastes such as rice or wheat straw, forestry residues and paper pulp from the paper industry. Food waste is another feedstock rich in functionalized molecules. Although it is biodegradable, it should be valorized as a raw material for renewable chemicals, materials and biofuels, leading us towards waste minimization and reduced dependence on fossil resources. The utilization of waste materials has the key advantages that it avoids competition for agricultural land that might be used for food production whilst generating value from residues that might otherwise go to waste. For these reasons, waste valorization is seen as an increasingly important source of both chemicals and energy.
In addition to the extractable functional molecules found in biomass, we can also make additional useful functional molecules or "platform molecules", such as succinic acid, lactic acid and levoglucosenone, by biochemical or thermochemical processing of the bulk cellulosic components of many types of biomass. A biorefinery is an analogue to the current petro-refinery in the sense that it produces energy and chemicals. The major differences lies in the raw material it will use, ranging from biomass to waste (Figure 1.2).
Biorefineries can be considered to belong to three types. Type 1 biorefineries focus on the conversion of one feedstock, using one process and targeting one product. A biodiesel production plant would be a good example: rapeseed or sunflower is used for oil extraction, which is subsequently transesterified to produce fatty acid methyl esters or biodiesel using methanol and a catalyst.
Type 2 biorefineries differ from the first type by the number of products. A typical example is the production of starch, ethanol and lactic acid together with high fructose syrup, corn syrup, corn oil and corn meal from corn wet mill operations. A more recent example that has been suggested is the use of citrus waste, such as orange peel (Figure 1.3).16
Type 3 biorefineries allow for a wider range of technologies to be combined. They also allow for a higher number of products generating two or more bio-based products and the residue is used to produce energy (either fuel, power and/or heat). Examples include whole crop biorefineries, which make use of several agricultural by-products originating from the same crop. Type 3 biorefineries are typically the ones targeting the production of chemicals and fuels.
As the biorefinery concept is developed, it is imperative that the use of clean technology is applied, ensuring its output(s) are truly sustainable. The IEA Bioenergy Task 42 defines biorefining as "the sustainable processing of biomass into a spectrum of bio-based products (food, feed, chemicals and/or materials) and bioenergy (biofuels, power and/or heat). In the future, various biorefineries will emerge commercially, taking advantage of flexible technology, helping the concept of a biorefinery to process locally available biomass in an integrated fuel-chemical-material-power cycle, improving the quality of life of the local population and lowering the environmental impact governed by the three dimensions of sustainability: environmental protection, social progress and economic development.
Green Chemistry is helping to drive the renewables revolution, pointing the way towards the substitution of fossil feedstocks and towards a more circular economy approach in resource utilization. The three fundamental stages in the product lifecycle are no longer enough — we now need to add a stage that returns the resources from the spent article to useful production. This could in principle be part of the natural carbon cycle for organic materials so that we only need ensure the articles are collected (better infrastructure) and that they are (quickly) biodegradable. The limitation is that nature tends to transfer most of its carbon in the form of carbon dioxide and other simple molecules, which then require effort to build up (by performing chemical reactions etc. that consume resources and generate their own waste). In the case of inorganic resources, we cannot rely on any sort of natural cycle for most elements. Our current linear approach of extracting ores, processing them to make metals, using those metals in the manufacture of complex articles, and then disposing of them in landfill sites cannot return the resources to us in any useful way. Rather we need to build up our own inorganic resource closed loop systems whereby the resources, typically metals are recovered from the original articles in which they are used, and in a form that can be easily used for the same or different application. This will require fundamental changes in the design of articles enabling easy disassembly at a resource level — sometimes referred to as "benign by design".
1.4 Major Initiatives Worldwide
As has been discussed, increasing legislation, limited resources, and changes in scientific and public opinion mean that there is a growing need for industries and academia to work together towards greener and more sustainable practices. It is vital that we equip the next generation of scientists with the knowledge and skills to do so.
(Continues...)
Excerpted from Green and Sustainable Medicinal Chemistry by Louise Summerton, Helen F. Sneddon, Leonie C. Jones, James H. Clark. Copyright © 2016 The Royal Society of Chemistry. Excerpted by permission of The Royal Society of Chemistry.
All rights reserved. No part of this excerpt may be reproduced or reprinted without permission in writing from the publisher.
Excerpts are provided by Dial-A-Book Inc. solely for the personal use of visitors to this web site.