Inorganic Chemistry of the Main-Group Elements: Volume 2 / Edition 1 available in Hardcover
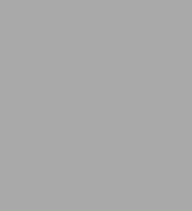
Inorganic Chemistry of the Main-Group Elements: Volume 2 / Edition 1
- ISBN-10:
- 0851867626
- ISBN-13:
- 9780851867625
- Pub. Date:
- 01/01/1974
- Publisher:
- RSC
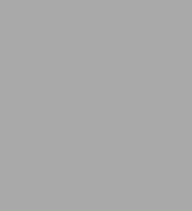
Inorganic Chemistry of the Main-Group Elements: Volume 2 / Edition 1
Hardcover
Buy New
$392.00-
SHIP THIS ITEMIn stock. Ships in 1-2 days. Not Eligible for Free ShippingPICK UP IN STORE
Your local store may have stock of this item.
Available within 2 business hours
Overview
Product Details
ISBN-13: | 9780851867625 |
---|---|
Publisher: | RSC |
Publication date: | 01/01/1974 |
Series: | Specialist Periodical Reports - Inorganic Chemistry of the Main-Group Elements , #2 |
Pages: | 740 |
Product dimensions: | 5.45(w) x 8.50(h) x (d) |
Read an Excerpt
Inorganic Chemistry of the Main-group Elements Volume 2
A Review of the Literature Published Between September 1972 and September 1973
By C. C. Addison
The Royal Society of Chemistry
Copyright © 1974 The Royal Society of ChemistryAll rights reserved.
ISBN: 978-1-84755-638-7
CHAPTER 1
Elements of Group I
BY R. J. PULHAM
1 Introduction
In this chapter individual references which are inter-related are grouped together to make a section and, therefore, reference to several alkali metals may feature in a single section. Each reference, however, appears once only within this chapter so that, if described in one section, it will not be duplicated in any other. Single references to topics are presented systematically in the section on the appropriate metal.
The elements of Groups I and II are so closely linked in some instances that a section describing them jointly is presented to avoid duplication in Chapter 2. Such a case is the section on 'Molten Salts', which covers the chemistry of the molten salts of both Groups I and II but is presented only in this chapter.
2 The Alkali Metals
The hyperfine structure of the 1 sn3P terms of singly ionized lithium –6, –7(Li II) has been investigated by the beam-foil technique. Zero-field quantum beats were observed in the intensity decays of transitions from the 1 sn3P terms (n = 2, 3, or 4) in Li II and the magnetic hyperfine coupling constant was determined for each isotope for the 2p3P terms. Preliminary values for the coupling constants are [MATHEMATICAL EXPRESSION NOT REPRODUCIBLE IN ASCII]. The measured fine structures agree within a few percent with recent ca1culations.l The Auger electron spectrum of freshly filed lithium contains an emission peak at 51.7 eV which is attributed to the KL1, L2, Auger transition, and unidentified peaks at 27.5 and 8eV. On exposure to oxygen the peaks at 51.7 and 27.5 eV disappeared but the 8 eV peak intensified. The low-energy spectrum was characterized by emissions at 13.3, 24.0, 33.0, and 40.0 eV due to Auger transitions of lithium, oxygen, and lithium monoxide. A value of 3.05 eV is reported for the work function of freshly prepared lithium films.
Lithium, the 'not so rare metal', is reviewed. The discussion covers the occurrence, production, and uses of the metal and its compounds. The metal has considerable potential in the future generation of electrical energy from the fusion reaction:
[MATHEMATICAL EXPRESSION NOT REPRODUCIBLE IN ASCII]
The supply of tritium for this process is derived from:
[MATHEMATICAL EXPRESSION NOT REPRODUCIBLE IN ASCII]
Within this context, the chemical, physical, and thermal properties of lithium that are related to its use in fusion reactors have been reviewed. These include natural abundance, thermodynamic and transport properties, characterization, analysis purity control, and corrosion of materials by the molten liquid. Problems associated with tritium in the metal are also covered, as is the separation of tritium from lithium by crystallization or diffusion. The pressures of hydrogen isotopes in equilibrium with their solutions in liquid lithium have been measured. The square root of the hydrogen pressure is proportional to the hydrogen concentration in accordance with Sievert's Law. Graphical data are presented for 2H. The metal is also chemically very reactive. The effect of temperature and pressure on the reaction of static molten lithium with oxygen, nitrogen, and the compounds CCl2F2, C4F8, and SF6 has been studied. The metal is heated inductively under vacuum and a small known volume of gas exposed to the surface. Pressure and temperature changes are followed by rapid-response instrumentation. The liquid metal is a versatile solvent for both non-metals and metals. Non-metals when dissolved in the metal may not have the same deleterious corrosive effect on containment materials as they do with sodium. Chemical processes are affected by the different thermodynamic stability of lithium compounds. This is illustrated by the effect of oxygen on the chemical corrosion of niobium and tantalum by static liquid lithium at 600 °C in capsules. An increase in the oxygen concentration of lithium from 100 to 2000 p.p.m. has no measurable effect, a result which is contrary to the effect of similar oxygen concentrations in liquid sodium or potassium. The free energy of formation of lithium oxide is so great that the liquid metal getters niobium and tantalum to an oxygen level ≤ 20 p.p.m. regardless of the oxygen concentration in the lithium. When the transition metals contain more than a threshold level of oxygen (400 and 100 p.p.m. for Nb and Ta respectively), chemical attack by lithium occurs at the grain boundaries with the formation of ternary compounds containing lithium, oxygen, and transition metal. Methods of analysing the liquid are obviously important. Photon activation appears applicable for the analysis of nitrogen and oxygen. These elements are determined by photon activation with a microtron as a y-ray source. The N is separated by distillation as ammonia and collected in sulphuric acid for activity measurements. Oxygen is rapidly separated by distillation as water for coincidence counting. Sensitivity is 2 × 10-5 wt% for each element.
In addition to its role in the fusion reactor, liquid lithium features prominently in solid-state batteries, an area which has been reviewed. Lithium and another element are usually separated by a solid or liquid electrolyte permeable to lithium ions, which migrate to form a compound with anions of the second element, thus driving electrons through the external circuit. The second element has been halogen, though this may be replaced by a compound, e.g. vanadium pentoxide. Present interest is in the chalcogens, and several lithium–chalcogen systems have been investigated with this use in mind. Equilibrium phases in the Li–S system on the sulphur side of Li2S (the only compound observed) are determined by using an unusual vapour-transport technique. By this means equilibrium compositions of the melts at various temperatures can be obtained by utilizing the transport of sulphur vapour from one melt to another. The Li–S phase diagram exhibits a large miscibility gap which extends from the monotectic composition, 65.5 mol % S, to almost pure sulphur (0.035 mol % Li) at the monotectic temperature 362 ± 3 °C. The m.p. of Li2S is 1365 ± 10 °C. This is largely corroborated by a second study which gives the miscibility gap from 63 to 98.8 mol % S, the monotectic at 364.8 °C, the m.p. of Li2S as 1372 °C, and a critical temperature for the miscibility gap of > 600 °C. An e.m.f. method using cells of the type Li|Li halide eutectic mixture|Li in selenium is used to determine thermodynamic quantities in the lithium-selenium system. From the cell data the standard free energy of formation of Li2Se at 360 °C is calculated as –94.0 kcal mol-1. In the Li–Te phase diagram, eutectics occur at 179.9 °C near the lithium axis at >99.0 atom % Li, 448.5 °C at 35.7 atom % Li, and 423.1 °C at 10.5 atom % Li. Two intermediate compounds are present, Li2Te and LiTe3, melting at 1204.5 and 459.9 °C, respectively.
The spectrum of doubly ionized sodium III has been studied at 2500 — 1300 Å and the analysis has been revised and extended as regards the 2p44s, 3p, and 3d configurations. The number of classified lines is now 177, out of which 110 are newly observed and classified. Some of the older classifications are altered and ca. 80 lines rejected as spurious. The following terms and levels are new: [MATHEMATICAL EXPRESSION NOT REPRODUCIBLE IN ASCII] configurations are now complete. In the range 380 — 180 A about 90 lines are measured, of which 50 are reported for the first time. The third, fourth, and fifth (i.e. Na IV, Na V, and Na VI) spark spectra of sodium have been re-photographed at 80 — 2400 Å and the measurements confirm earlier analyses. New lines in Na IV, Na V, and Na VI are observed for the first time and classified. The K X-ray spectra of sodium excited by protons, helium, and oxygen ions of 0.8, 3.2, and 30 MeV, respectively, have been measured. The strongest lines are the normal Kα satellite spectra produced by multiple electron vacancies in single ion — atom collisions. In the H- and Heion-induced spectra, Kα1,2 is the strongest transition. On the absorption side, the spectrum of atomic sodium between 30 and 150 eV shows lines which can be attributed to the excitation of a 2s or 2p electron. Considerably broad and asymmetric absorptions above the 1P1 series limit are due to the simultaneous excitation of a 2p and 3s electron.
The electrical conductivity of sodium vapour has been measured in a coaxial-cylinder, two-electrode system at 827 — 1227 °C. The results support the conductivities calculated by E. J. Robbins et al. (1967, 1968) on the basis of a model for the vapour consisting of Na1, Na2, and Na4 moieties. The concentration of multi-atom associates in saturated vapours at various temperatures can be semi-empirically derived. In the case of unsaturated vapours, the number of associates tends to zero with increasing size. The symmetry of the associates, binding energies, and mobility for sodium and potassium are given. The effect of temperature is calculated on the equilibrium between the concentration of free sodium atoms and those combined in the cluster. Agreement with experimental data is satisfactory. The best available thermodynamic data on liquid metals are tabulated and include m.p., entropies of fusion, heats of fusion, and heat capacities. Graphical correlations are presented between heats of fusion and melting points, and between entropies of fusion and structural parameters. Heat-capacity anomalies are discussed in terms of the electron configuration of the metal. The surface tensions of molten alkali metals from their melting temperatures up to 1127 °C have been determined in a special high-temperature, high-pressure apparatus. The surface tensions/dyn cm-1 as a function of temperature (t/°C) under an atmosphere of their own vapours are given by:
[MATHEMATICAL EXPRESSION NOT REPRODUCIBLE IN ASCII]
The values correlate well with those previously published.
As with lithium, the majority of the literature on the commercial uses of metallic sodium is devoted to aspects of the generation of electrical energy either where the metal is used as a coolant in fast nuclear reactors or used as an electrode in high-power batteries. An indication of the extent of the nuclear use of liquid sodium is provided in a review of the principal programmes involving fast reactors in the world. Technological aspects are also represented. These applications steadily reveal new chemical properties of sodium and its compounds. This is illustrated in the proceedings of a conference on the Liquid Alkali Metals which covers fundamental chemistry, physics, analytical and instrumentation techniques, sodium-water reactions, carbon and fission-product behaviour in sodium, physical processes, corrosion, and mass transfer. Also, chemical reactions in liquid alkali metals are discussed, with particular emphasis on solvation aspects. A comparison is made of the nature and properties of liquid metals, representing continuous reaction media, with other non-aqueous solvents, e.g. molecular liquids, representing discontinuous media. Chemical aspects are generally found in the purification, analysis, and corrosion areas. The non-metals oxygen, hydrogen, nitrogen, and carbon, when dissolved in the liquid metal, have a deleterious effect on transition metals, which are invariably employed as containment materials. Purification and analytical techniques, therefore, are primarily designed to remove and monitor- these elements, in many cases in situ. To prevent nitriding and embrittlement of steel submerged in liquid sodium, ca. 1 atom % calcium or magnesium can be added to the liquid. These metals, with their strong chemical affinity for nitrogen, effectively isolate the steel from nitrogen. To analyse for hydrogen in liquid sodium, a nickel thimble is immersed in the liquid and evacuated to 10-6 — 10-8 Torr on the inside. The process relies on the equilibrium between dissolved and gaseous H. Hydrogen leaves the liquid, diffuses through the nickel, and establishes an equilibrium pressure, the magnitude of which is dependent on its concentration in the liquid. As little as 0.02 ± 0.01 p.p.m. of hydrogen can be detected. Hydrogen may exist in a sample of sodium in several forms, i.e. dissolved sodium hydride, solid sodium hydride, or sodium hydroxide. To distinguish between these requires several processes. All the hydrogen is released as gas by vacuum fusion in a bath of tin at 350 °C. Amalgamation of the sample, however, releases only dissolved hydrogen. Subsequent heating to 200 °C decomposes solid sodium hydride. The remaining hydroxide hydrogen may be determined by difference. Alternatively, the remaining sodium amalgam is heated in an argon stream at 400 °C. Under these conditions sodium reacts with sodium hydroxide to give hydrogen, NaH, and Na2O. Hydride decomposes to give hydrogen, which is determined by gas chromatography. Hydrogen is also soluble in liquid potassium. Over the temperature range 340 — 440 °C, the solubility is given by the equation:
log(C/p.p.m. by wt.) = 6.8 – 2930/(T/K)
The pressure of hydrogen in equilibrium with the saturated solution of hydrogen in the metal is given by:
log(P/Torr) = 11.3 – 5860/(T/K)
These pressures are the dissociation pressures of potassium hydride according to:
KH = K + ½H2
The enthalpy of formation, ΔHo, of potassium hydride as derived from these pressures is – 13.7 kcal mol-1. The equilibrium pressures of hydrogen above unsaturated solutions of the gas in the metal are given by:
P1/2 = C × 104/14.2
where C is in weight %. Thus Sieverts' Law is obeyed (P1/2 [varies] C), which indicates that the species of hydrogen in the metal is monatomic.
Most interest has centred on solutions of oxygen in liquid sodium since this element, more than any other, renders the liquid metal corrosive. Vanadium, niobium, and tantalum, and their alloys, have a low intrinsic solubility in liquid sodium and suffer but slight corrosion. The presence of oxygen in the liquid, however, leads to penetration by non-metals into the transition metal, internal oxidation, oxide scale formation, spallation or dissolution of oxides, and, in some cases, penetration by the sodium. Whether the transition-metal surface oxidizes or whether sodium extracts the oxygen contained in or on the metal depends largely on the relative free energies of formation of the transition-metal oxide and sodium oxide, respectively. The situation is more complicated, however, since the energy balance is affected by the activity (or concentration) of oxygen in the sodium or in the solid metal, i.e. a dilute solution of oxygen in liquid sodium may be reducing whereas a more concentrated solution will oxidize a particular transition-metal surface. Further complications arise when ternary compounds form which are stable in sodium. Most transition metals form at least one ternary oxide with sodium. These points are illustrated below. Vanadium, exposed at 600 °C to static sodium solutions containing oxygen up to 4000 p.p.m., getters all oxygen from solutions which contain less than 2000 p.p.m. The distribution coefficient for oxygen between vanadium and sodium is greater than 10 at 600 °C. By alloying chromium or molybdenum with vanadium, the activity coefficient of oxygen in the solid alloy is increased and hence the solubility is reduced. In sodium containing 2000 p.p.m. oxygen at 600 °C, alloys of vanadium containing titanium or zirconium form internal precipitates of oxide during the gettering, and the concentration of oxygen dissolved in the alloy approaches that of the same alloy without titanium or zirconium. When titanium and zirconium are immersed in liquid sodium containing dissolved sodium oxide at 600 °C, the surfaces are covered with the ternary oxides Na4TiO4 and Na2ZrO3, respectively. These compounds were identified in situ by their V-ray diffraction patterns. The compound Na4TiO4 was detected when the sodium contained from 100 to 12 000 p.p.m. oxygen. At the end of long contact times the oxide TiO formed below the ternary oxide, which suggests that the ternary oxide is formed first and is followed by diffusion of oxygen into the substrate metal to form TiO. With zirconium, a rapid formation of the oxide ZrO is postulated which is followed by a slow reaction with dissolved sodium monoxide to give Na2O, ZrO2. Liquid potassium, like sodium, also becomes more corrosive towards transition metals when it contains dissolved oxygen. Analysis of potassium after immersion of tantalum at 600, 800, and 1000 °C shows that the amount of tantalum finding its way into the alkali metal increases with the amount of oxygen originally dissolved in the liquid metal. Again, a ternary oxide phase is formed. Oxygen held in the tantalum also promotes corrosion when the transition metal contains more than a threshold concentration of oxygen in solid solution; potassium penetrates the solid metal intergranularly and transgranularly via ternary oxide formation. The threshold levels of oxygen for this type of attack at 600, 800, and 1000 °C are 500, 700, and 1000 p.p.m., respectively. Distribution of radioactive corrosion products is obviously important in flowing sodium. Particulate material deposits according to flow rate and geometry of circuit, size of particulate, and whether the species is soluble in the sodium or reacts preferentially with metallic parts of the circuit. Initial experiments have investigated the transport and deposition characteristics of 59Fe, 54Mn, and 60Co. The 59Fe behaviour is characterized by its appearance as a firmly adherent layer on pipework downstream of the test section. 60Co is similar to iron but the deposit is less strongly attached. The behaviour of 54Mn is characterized by its rapid and highly preferential migration to the coldest part of the circuit. Adsorption of caesium, a product of the fission process, also occurs from solution in sodium at transition-metal surfaces. Between 100 and 200 °C, caesium is adsorbed on to nickel and steel (EN-58B) surfaces but at 800 °C the adsorption is eliminated The mechanism of adsorption is not clear.
(Continues...)
Excerpted from Inorganic Chemistry of the Main-group Elements Volume 2 by C. C. Addison. Copyright © 1974 The Royal Society of Chemistry. Excerpted by permission of The Royal Society of Chemistry.
All rights reserved. No part of this excerpt may be reproduced or reprinted without permission in writing from the publisher.
Excerpts are provided by Dial-A-Book Inc. solely for the personal use of visitors to this web site.
Table of Contents
Contents
Chapter 1 Elements of Group I By R. J. Pulham, 1,Chapter 2 Elements of Group II By R. J. Pulham, 73,
Chapter 3 Elements of Group III By G. Davidson, 103,
Chapter 4 Elements of Group IV By P. G. Harrison and P. Hubberstey, 225,
Chapter 5 Elements of Group V By A. Morris and D. B. Sowerby, 430,
Chapter 6 Elements of Group VI By M. G. Barker, 560,
Chapter 7 The Halogens and Hydrogen By M. F. A. Dove, 650,
Chapter 8 The Noble Gases By M. F. A. Dove, 676,
Author Index, 684,