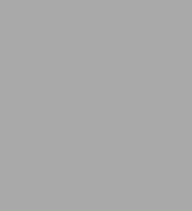
Materials Challenges: Inorganic Photovoltaic Solar Energy
355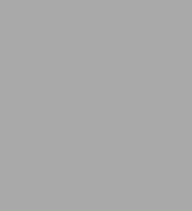
Materials Challenges: Inorganic Photovoltaic Solar Energy
355Hardcover
-
SHIP THIS ITEMIn stock. Ships in 1-2 days.PICK UP IN STORE
Your local store may have stock of this item.
Available within 2 business hours
Related collections and offers
Overview
Product Details
ISBN-13: | 9781849731874 |
---|---|
Publisher: | RSC |
Publication date: | 12/09/2014 |
Series: | Energy and Environment Series , #12 |
Pages: | 355 |
Product dimensions: | 6.30(w) x 9.30(h) x 1.10(d) |
About the Author
Read an Excerpt
Materials Challenges
Inorganic Photovoltaic Solar Energy
By Stuart J. C. Irvine
The Royal Society of Chemistry
Copyright © 2015 The Royal Society of ChemistryAll rights reserved.
ISBN: 978-1-84973-187-4
CHAPTER 1
Introduction and Techno-economic Background
STUART J. C. IRVINE AND CHIARA CANDELISE
1.1 Potential for PV Energy Generation as Part of a Renewable Energy Mix
Climate change became one of the major drivers for changing the balance of energy generation and supply in the latter part of the 20th century and the beginning of the 21st century. The increase in carbon dioxide (CO2) concentration in the atmosphere over the past century to a figure approaching 400 parts per million (ppm) is taking it closer to the historical 450 ppm concentration where there was virtually no ice on the planet. The Intergovernmental Panel on Climate Change (IPCC) has set a maximum increase in global temperature of 2 °C which Hansen et al. argue can only be achieved if atmospheric CO2 falls to 350 ppm to avoid irreversible loss of the ice sheet. Meinshausen et al. 2 put a figure on cumulative CO2 emissions into the atmosphere of 1000 Gt between 2000 and 2050 would yield a 25% probability of exceeding the 2 °C threshold in global warming.
The world electricity supply is heavily dependent on coal, gas and oil, accounting for 62% of the total for Organisation for Economic Co-operation and Development (OECD) countries in the period January to April 2012, according to the International Energy Agency (IEA). In the same period the balance was made up of 19% nuclear, 14% hydro and a mere 5% for other renewable energy such as wind, solar and geothermal. However, this small contribution from renewable energy has been increasing and was up by 1% on the same period the previous year. Vries et al. have analysed the potential mix of wind, solar and biomass (WSB) to 2050 and concluded that this could be achieved at an energy cost of 10 US cents per kWh of energy, displacing fossil fuel electricity generation.
Although the annual growth of the photovoltaic (PV) sector has been in the range of 30 to 40% over the past 20 years, it is still at an early stage of potential development both in terms of capacity and price. A number of different scenarios exist to predict the future renewable energy mix that will displace combustion of fossil fuels. For example, the World Business Council for Sustainable Development (WBCSD) predicts 50% electricity generated from renewable energy sources by 2050 with 15% generated by solar PV. Other scenarios give a range for renewable energy generation from 31% from the IEA to a number of studies predicting 50%, including the German Advisory Council on Climate Change, Greenpeace and Shell's sustainable development plan. All the scenarios consider PV solar energy to be a significant part of the energy mix though the extent of penetration into the energy mix changes according to the different scenarios. Looking beyond 2050 the proportion of renewable energy and in particular PV solar energy will continue to grow and the German Solar Industry Association predicts that the proportion of PV solar electricity generation will increase to over 50% of the mix by the end of the century. In a separate study by Fthenakis et al. which looked at the potential for combined PV and concentrator solar power (CSP) in the USA, it was predicted that all the electrical energy could be produced from the Sun combined with compressed air energy storage.
In 2011 over 25 GW of PV was installed worldwide, taking the cumulated PV installations to over 50 GW. Most of these installations are based on crystalline silicon (c-Si), but the share of thin ?lm PV has grown over the past decade and currently stands at between 10 and 15%.
In terms of climate change there is a carbon cost in manufacture based on the dependence of electricity used in PV module manufacture on fossil fuel sources. The melting of silicon to form the c-Si requires a temperature of over 1400 °C. In contrast, thin film PV uses processing temperatures below 600 °C and therefore will require less energy. Pehnt carried out a lifecycle analysis of c-Si PV module manufacture. With the current German energy mix, where there is 566 g of CO2 per kWh of electricity, this leads to an emission of 100 g of CO2 equivalent per kWh of electricity generated over the lifetime of the PV module. As the proportion of non-fossil fuel energy sources in the energy mix increases this could be halved to 50 g of CO2 equivalent per kWh. This compares with around 10 g of CO2 equivalent per kWh for onshore wind and 1.5 MW hydropower. Other factors that will reduce this carbon emission are the efficiency of solar energy conversion and processing temperatures. Thin film PV is currently less efficient than c-Si, roughly 10% compared with 15% but the process energy per square metre is less, which leads to an overall reduction in CO2 emission. Fthenakis et al. estimated that less than 20 g CO2 equivalent per kWh was emitted for 9% efficient cadmium telluride modules.
Figure 1.1 illustrates that cadmium telluride (CdTe) thin film PV is very competitive in terms of environmental emissions compared with other technologies. Recent improvements in efficiency in thin film CdTe modules to more than 12% would reduce carbon emissions to less than 15 g CO2 equivalent per kWh. From these estimates it is clear that the adoption of thin film PV modules will make an impact on reducing carbon emissions in PV module manufacture.
In this book we examine the materials challenges for inorganic thin film PV that will influence both the environmental impact and the economic payback, as discussed later in this chapter.
1.2 Historical Development of Thin Film PV
Observation of the photovoltaic effect goes back to Becquerel, first published in 1839. However, practical devices were only realised with the development of high purity silicon for semiconductor devices and the first demonstration of a silicon PV cell at Bell Labs in 1954.10 The initial devices had a conversion efficiency of 6%, but this rapidly improved and established silicon solar cells as a source of power for the early satellites. By 1980 c-Si cells had reached 16% AM1.5 (air mass) efficiency and have continued to improve to the present day with record efficiency of 25%.
Although the global PV market is dominated by c-Si, there has been rapid growth of other PV cell and module technologies that offer a very wide choice of PV materials, each with its potential advantages and disadvantages compared with the silicon benchmark. For c-Si, the development of cast multi-crystalline silicon has provided a cheaper alternative to single crystal silicon with only a small penalty in loss of efficiency. By 2004 multi-crystalline silicon single cell record efficiency had exceeded 20%.
The earliest thin film PV dates back to the late 1960s with the emergence of amorphous silicon (a-Si) on glass substrates as a much cheaper and lower energy option than the crystalline silicon cells. The first a-Si solar cell reports date back to 1976 but have never achieved the efficiency of the c-Si counterparts. This is discussed in more detail regarding the fundamental properties in Chapter 2 and in further detail in Chapter 3. In many respects, the history of a-Si has established a cheaper alternative to c-Si and led the way for the emergence of other thin film materials. The techno-economic trade-off between cost of manufacture and module efficiency is discussed later in this chapter and provides a context for the remainder of the book. Single junction a-Si cells have now achieved over 10% stabilised efficiency and over 12% when combined in a tandem cell with a micro-crystalline junction. Early applications of a-Si solar cells were seen in consumer products where the low cost and monolithic integration were important but longer term stability was not as important as for larger scale power applications. Monolithic integration of a-Si onto glass has enabled a range of architectural applications to be explored that would have been difficult or impossible to achieve with c-Si.
The origins of CdTe PV cells goes back to a Cu2Te/CdTe cell reported in 1976 by Cusano. This sparked a rapid increase in the possibilities for compound semiconductor thin film PV that included, around the same time, the first interest in the Chalcopyrite structure of copper indium diselenide (CIS) with the work of Wagner et al. on single crystal material and Kazmerski et al. on thin film PV. Further developments on CIS thin film PV led to alloying with gallium to form CIGS where indium can be substituted with gallium to change the bandgap of the absorber. For both CIGS and CdTe cells the absorber is p-type and the preferred n-type heterojunction material has become cadmium sulphide. Although early commercialisation of thin film PV was with a-Si, the more complex polycrystalline chalcogenides have shown the potential to achieve higher module efficiencies and good long-term stability. Large-scale thin film module manufacture of CdTe and CIGS modules has been demonstrated by First Solar and Solar Frontier, respectively. The significance of manufacturing volume in the cost of module manufacture is discussed in Section 1.4 along with the significance of continual improvements of module conversion efficiency.
The past 20 years have seen the development of many alternative materials and designs for PV solar cells suitable for a range of different applications. The drive for low cost materials and low temperature processing has generated a huge amount of research in dye sensitised solar cells (DSC) and organic photovoltaics. The DSC owes its origins to photoelectrochemical cells and the origins of this go back to Becquerel. The principal of these cells is the release of an electron from a suitable dye such as ruthenium organometallics into the conduction band of anatase TiO2. The dye is regenerated from the counter electrode with an iodide redox couple and is shown schematically in Figure 1.2. The highest efficiency achieved for laboratory cells is achieved 11%. Commercial exploitation of this highly manufacturable process has been demonstrated by G24i Power amongst others. In the case of G24i Power the DSC is deposited onto a plastic sheet in a roll to roll process. These cells perform particularly well under low light intensity and are being marketed for consumer products.
A further development of organic semiconductors as an alternative to their inorganic counterparts was the formation of polymer blends that can separate the excitons formed when light is absorbed in organic semiconductors. This is essentially a room temperature process and represents a very low carbon footprint. The excitons are strongly bound so separation of the electron–hole pair is not as easy as with inorganic semiconductors and can only occur at the interfaces. This is made more difficult by the short excitonic diffusion length which is around 10 nm. Hence the success of polymer blends which create a large interface area between the electron donor and electron acceptor polymers to improve collection efficiency. These cells have shown rapid progress over the past 10 years with only 3% efficient cells in 2002 rising to 10% in 2012. These cells are still very much in the research phase, but are likely to be part of the PV materials mix in future generations of PV device applications.
The highest efficiency PV devices are based on III–V epitaxial materials. The highest efficiency single junction cell is not c-Si, as one might expect, but GaAs with a world record efficiency of 28.3%, not too far off the Shockley–Queisser limit described in Chapter 2. These high efficiency cells require very high quality epitaxial layers, which are achieved through lattice matching of each of the layers in the structure. By lattice matching to Ge substrates, it is possible to produce a triple junction of lattice matched layers of InGaP and InGaAs to cover the blue and red parts of the spectrum, respectively, with the infrared covered by a junction formed with the Ge substrate. These triple junction cells have been developed as high performance solar cells for space but there is now increasing interest for use with concentrators (lenses or reflectors) for terrestrial power generation. The III–V cells are covered in greater detail in Chapter 8. The efficiency of these cells has been increasing rapidly in the past 10 years going from 35% in 2002 to 41.6% in 2009 for around 500 suns concentration. In December 2012, Solar Junction beat its own previous world record of 43.5% to achieve a National Renewable Energy Laboratory (NREL) verified efficiency of 44% at 947 suns. This shows the same kind of rapid increase over the past decade as the newer organic PV at the other end of the efficiency scale and reflects the advanced semiconductor engineering of epitaxial III–V semiconductors. The challenge for thin film PV moving forward is to find a similar surge in performance. This book explores new developments that could lead to such rapid progress for thin film amorphous and polycrystalline PV.
1.3 The Role of Inorganic Thin Film PV in the Mix of PV Technologies
There is now a wide variety of PV materials and devices that appear to be competing for the same space of low cost per Watt peak (Wp). Conibeer has described the generations of PV devices according to their potential to reduce the cost per Wp. This is reproduced in Figure 1.3 showing the expected trend lines for the three generations of PV materials. The first generation is the currently dominant crystalline silicon cells which are relatively high cost but also high efficiency single junction cells. The second generation is the thin film inorganic monolithic modules that are much cheaper to produce per square metre but are also lower efficiency than the crystalline silicon cells. Both first and second generations are single junction cells which have a theoretical upper limit as explained in Chapter 2. The third generation seeks to breach this single junction limit through either multi-junction cells or other devices that can capture the energy from hot electrons. The cost per square metre is similar to the second generation but achieves a lower cost per Wp through gaining higher efficiency.
Organic PV (OPV) cells are also sometimes referred to as third generation. These can be single or multi-junction but offer the potential for even lower cost per square metre than thin film inorganic PV, largely because of lower deposition and processing temperatures. The limits to OPV efficiency are not understood in the same way as for inorganic PV so how far OPV can go in terms of low cost and high efficiency is not known at this point.
Inorganic thin film PV bridges between second and third generation PV. The cost per square metre is less than for crystalline silicon but has the potential to achieve the same single junction efficiency. There is also the potential for inorganic thin film PV to incorporate third generation PV concepts to achieve higher efficiency. In this respect the way has already been shown with III–V triple junction cells, but these are high quality single crystal and to truly achieve the potential of third generation PV this would have to be achieved with the lower cost fabrication of inorganic thin films.
Inorganic thin film PV has had to compete with a more mature and larger scale c-Si PV industry, but this scale is now being achieved by some of the thin film PV manufacturers and the significance of this is discussed in the next section. There are other aspects of inorganic thin film PV that can make this class of solar materials attractive over crystalline silicon. The absorber materials are direct bandgap semiconductors so requiring far less material to absorb the available solar radiation than for c-Si. This property is described in Chapter 2. Another advantage is the temperature coefficient of efficiency whereby the efficiency of all PV devices will decrease as the modules get hotter, but the coefficient for thin film PV is approximately half that of c-Si so will have advantages when being operated in hotter climates. Finally the monolithic integration of cells in a thin film module gives greater flexibility over the appearance of the module, making it look more uniform and giving it aesthetic advantages over c-Si modules for building integrated applications.
An example of the potential for thin film PV in architectural design is shown in Figure 1.4, which shows the 85 kWp CIS array on the technology centre of the OpTIC building in St Asaph, North Wales. The curved façade effect is actually created from a series of flat panels and strings are aligned with panels of similar elevation to avoid losses as the elevation of the Sun changes during the day. This façade has been in operation now for 10 years and the CIS thin film panels continue to perform well with similar output to their initial performance.
(Continues...)
Excerpted from Materials Challenges by Stuart J. C. Irvine. Copyright © 2015 The Royal Society of Chemistry. Excerpted by permission of The Royal Society of Chemistry.
All rights reserved. No part of this excerpt may be reproduced or reprinted without permission in writing from the publisher.
Excerpts are provided by Dial-A-Book Inc. solely for the personal use of visitors to this web site.
Table of Contents
Fundamentals of inorganic solar cells;Thin film silicon solar cells;
TCOs;
Cadmium telluride solar cells;
CIS, CIGS, Chalcogenides;
New chalcogenides;
III-V solar cells;
Nanomaterials;
Light Capture;
Photon management materials;
Conclusions and future developments;
Index;