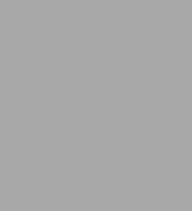
Natural Product Chemistry for Drug Discovery
458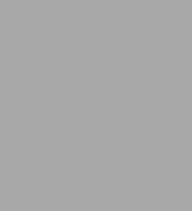
Natural Product Chemistry for Drug Discovery
458Hardcover(Edition. ed.)
-
PICK UP IN STORECheck Availability at Nearby Stores
Available within 2 business hours
Related collections and offers
Overview
Product Details
ISBN-13: | 9780854041930 |
---|---|
Publisher: | RSC |
Publication date: | 10/21/2009 |
Series: | RSC Biomolecular Sciences , #18 |
Edition description: | Edition. ed. |
Pages: | 458 |
Product dimensions: | 6.10(w) x 9.30(h) x 1.20(d) |
About the Author
Read an Excerpt
Carbohydrate Chemistry Volume 35
A Review of the Literature Published between January 2004 and December 2008
By Amelia Pilar Rauter, Thisbe K. Lindhorst
The Royal Society of Chemistry
Copyright © 2009 The Royal Society of ChemistryAll rights reserved.
ISBN: 978-1-84755-970-8
CHAPTER 1
Anomeric effects in pyranosides and related acetals
Sandrine Gerber-Lemaire and Pierre Vogel
DOI: 10.1039/b905496k
The anomeric effect, originally defined as the preference of an electronegative substituent at the anomeric position of pyranosides to stand in an axial rather than an equatorial position, results from the combination of multiple steric, stereoelectronic and medium interactions. A more generalized definition of the enthalpic anomeric effect refers to the gem-dioxy stabilizing effect which makes acetals more stable than their 1,n-dialkoxyalkane isomers (n > 1). This effect is far more important than the difference in stability between conformers of acetals as those found in axial and equatorial pyranoses and pyranosides. This review gives an up-dated view of enthalpic, conformational and also kinetic anomeric effects in pyranosides. The latter manifest themselves during heterolytical processes occuring at the anomeric centers of pyranosides and analogous compounds.
1 Introduction
Known for alkyl pyranosides since 1905 (e.g.: alkyl [apha]-D-glucopyranosides being more stable than their β-anomers) and rediscovered in 1955 by Edward, and by Lemieux and Chü in 1958, the conformational anomeric effect designs the contrasteric effect observed in acetals which renders the more sterically encumbered gauche/gauche conformers more stable than their anti/gauche and anti/anti conformers. Following the early interpretation of Lemieux based on electrostatic interactions, several explanations have been advanced to account for the origin of the conformational anomeric effect. This review presents the different models that provide insights in the understanding of anomeric effects.
2 The enthalpic anomeric effect
Acetals are more stable than their 1,n-dialkoxyalkane isomers (n > 1). Examples (Table 1) are given by comparing the standard heats of formation (gas phase) of 1,2-diethoxyethane (ΔHf° = -98.06 [+ or -] 0.25 kcal/mol) with that of 1,1-diethoxyethane (ΔHf° = -108.4 [+ or -] 0.74 kcal/mol), of 1,3-diethoxypropane (ΔHf° = -104.3 kcal/mol) with that of 2,2-diethoxypropane (ΔHf° = -121.1 kcal/mol), or of 1,4-dioxane (ΔHf° = -75.4 kcal/mol) with that of 1,3-dioxane (ΔHf° = -80.9 kcal/mol).
The gem-dioxy substitution stabilizing effect is called the "enthalpic anomeric effect". If one takes alkanes as reference compounds, one finds that exchange of a CH2–CH2 group by a CH(Me) group (isomerization) corresponds to a decrease of heat of formation of ca. -2 kcal/mol (for systems not affected by significant changes in gauche interactions). In the case of n, n + 1-dialkoxyalkanes isomerizing into n,n-dialkoxyalkanes, the change associated with the exchange of a RO–CH2–CH2–OR group with a (RO)2CH(Me) group amounts to -10 to -11 kcal/mol, which is about -8 to -9 kcal/mol more negative than for alkanes. This difference is a stabilization effect associated with gem-dialkoxydisubstitution. Interestingly in the case of dichlorides and dicarbonitriles, gem-disubstitution leads to destabilizations of ca. +2 and +8 kcal/mol, respectively (Table 1). The stabilizing gem-dioxy substitution effect is not always the same. It depends on the nature of the acetal. Beckhaus et al.proposed the following correction ΔΔHanom for the anomeric effect (ΔΔHanom = [ΔHdioxy - ΔHoxy] - [ΔHoxy - ΔH alkyl ]) (Fig. 1) to be applied to the additivity rule which allows estimation of heats of formation.
Methyl substitution of n-alkanes introduces a change in the standard heats of formation [ΔH°f (gas)] of -7 kcal/mol [exchange of a CH2 group for a CH(Me) group]. In the case of CH3CH2OMe, becoming (CH3)2CHOMe, ΔΔH°f= -8.5 kcal/mol and for 1,3-dioxane, methyl substitution at C(2) is accompanied with a more negative change of -11.6 kcal/mol. Even more dramatic are the 4-methyl and 2-methyl substituent effects on the standard heats of formation of 1,3-dioxane that reach -9.6 and 14 kcal/mol, respectively (Fig. 2).
A strong stabilizing geminal disubstitution effect is also observed for difluoro disubstituted hydrocarbons (e.g.: 2MeF [??] CH2F2 + CH4, ΔH°r = -12.3 kcal/mol; 2MeOMe [??] (MeO)2CH2 + CH4, ΔH°r = -13 kcal/mol) while dibromo and dichloro derivatives (e.g.: 2MeCl [??] CH2Cl2 + CH4, ΔH°r = -0.2 kcal/mol) do not benefit from this effect. The origin of this enthalpic anomeric effect is essentially an electronic one. Considering the two oxy or two fluoro substituents as two dipoles, electrostatic theory predicts the existence of families of geometries leading to a stabilization (Eel< 0). This phenomenon can be modeled by the relationship given in Fig. 3 for an ensemble of two positive charges q+ and two negative charges q-. The attractive interactions between negative and positive extremities of two different dipoles overcome the repulsing interactions resulting from the juxtaposition of two positive extremities of the two dipoles and from the repulsion between the two negative extremities of the dipoles.
[MATHEMATICAL EXPRESSION NOT REPRODUCIBLE IN ASCII]
Balance between repulsive and attractive electrostatic effects depends on charges q+,q- (electronegativity differences of the C–X bonds) or the nature of the dipole (type of bonds) and their orientation. In the case of acetals, one needs to consider an ensemble of not only two dipoles but of, at least, four (Fig. 4).
As we shall see, the conformational anomeric effects can be assigned to stability difference between the various conformers that this ensemble of dipoles can adopt. As a general rule, the latter enthalpy differences are much smaller than the enthalpic anomeric effects (gem-dioxysubstitution effects) defined above.
3 The conformational anomeric effect
The conformational anomeric effects design the contrasteric effects observed in acetals which render the more sterically encumbered gauche/ gauche conformers more stable than their anti/gauche and anti/anti conformers. Such effects were first evidenced by Jungins in 1905 and rediscovered by Edward in 1955 and by Lemieux and Chiu in 1958. They observed the higher stability of alkyl α-D-glucopyranosides in comparison with their β-anomers (Fig. 5).
Following these first discoveries, it was soon established that the conformational anomeric effect is not restricted to carbohydrates but applies to fragments of type R–X–A–Y where X stands for an atom bearing lone pairs, A represents an atom of intermediate electronegativity (i.e. C, P, S) and Y is an atom of higher electronegativity than A (i.e. N, O, halogens). These systems display a preference for the substituent on the anomeric carbon to adopt a synclinal (gauche) position rather than a anti-periplanar (anti) position (Fig. 6).
Consequences of the conformational anomeric effect are largely expressed in monosaccharides and their derivatives. One recognizes the conformational endo-anomeric effect for pyranosides with a polar X group at C(1) (contrasteric electronic stabilization effect: Fig. 7A) and conformational exo-anomeric effect for glycosides (acetals) in which the alkyl group of the exocyclic moiety is synclinal (Fig. 7B, C).
3.1 Conformational endo-anomeric effect in hexoses
In many monosaccharides the proportion of the α-anomer in aqueous solution is higher than the expected ratio only based on steric inteactions. In particular, while the predicted ratio in aqueous solution at 25 °C based on the A value for axial vs.equatorial cyclohexanol is 11/89, the observed ratio for D-glucose is 36/64 for α-pyranose/β-pyranose. Based on quantitations by 13C-NMR, Serianni et al. disclosed the ratios of the different forms of aldohexoses in water (Table 2).
The authors also evidenced an increase of the percentages of α-talopyranose and α-talofuranose upon 2H substitution at C(1) concomitantly with a decrease of the percentages of β-conformers. This observed shift on the talopyranose anomeric equilibrium was attributed to the preference of the shorter C–2H bond (relative to the C–1H bond) to assume an equatorial orientation.
3.2 Theories for conformational anomeric effects
The first interpretation of the conformational anomeric effect, given by Edward, invoked more favorable electrostatic interactions in the axial anomers than in the equatorial anomers of carbohydrates (Fig. 8).
For instance, in structure 12-e, the C–X and C–O dipole moments are additive, leading to a destabilization of the molecule by increasing the energy. In structure 12-a, offset of the C–X and C–O dipole moments minimizes electrostatic interactions, thus leading to a more stable conformation. This electrostatic model was supported by the observed increase of the percentage of the equatorial conformation of 2-methoxy tetrahydropyran (14) when moving from a non-polar to a polar solvent (Table 3). In this model, the polar groups are not polarizable and lead to dipole/dipole (hard/hard) interactions.
The decrease of the anomeric effect in polar solvents was also supported by quantum mechanics calculations. Nevertheless further studies on the anomeric effect demonstrated the limitations of the electrostatic model. In particular, Juaristi et al. demonstrated that, at low temperature, the dependence of conformational equilibria of 2-carbomethoxy-1,3-dithiane upon solvent shows an opposite trend to the stronger anomeric effect in less polar media observed at 25 °C (Table 4).
Similar observations have been reported by Pinto for the conformational equilibrium in 2-[(4-methoxyphenyl)seleno]-1,3-dithiane (Fig. 9). Although, at 273 and 300 K, the proportion of the more polar equatorial isomer 17 increases as the dielectric constant of the medium increases, no apparent correlation exists at low temperature.
The rationalization of the conformational anomeric effect solely based on electrostatic interactions fails to account for these solvent effects. Another interpretation based on bond polarizability in 1,1-dialkoxyalkyl systems calls electronic transfer from a non bonding electron pair of one oxygen atom to the empty σ*C–O orbital from the other alkoxy substituent (Fig. 10).
In this model, one considers the acetals to be composed of polarizable dipolar moieties that can be stabilized by electron transfer from an electron- rich moiety (non-bonding electron on oxygen; low ionisation energy) to adjacent polar and polarizable moieties (high electron affinity). A strong overlap between n(O) and σ*C–O orbitals optimizes this electronic transfer. As these orbitals are not spherical, n(O)/ σ*C–O overlap depends on conformation (torsional angle θ, Fig. 6 and 7). For alkyl pyranosides, the electronic transfer n(O) -> σ*C–O involving the intracyclic oxygen atom with the C(1)–OR bond is more favorable in axial conformers (conformational endo-anomeric effect). This theory predicts also that rotamers in which the R group and the O(5) oxygen atom are gauche should be favored over rotamers presenting the R group and the intracyclic O(5) oxygen atom in antiperiplanar relationship (Fig. 10). Manifestation of this exo-anomeric effect is given by a clear difference in C–O bond lengths. In equatorial pyranosides (synclinal, eq-exo-syn, Fig. 7C), the intracyclic C–O bond is longer than the exocyclic C–O bond.
At that stage, a correct understanding of the non-bonding electron pairs of oxygen (and other heteroatoms involved in conformational anomeric effect) is of crucial importance. In many instances, a sp3-hybridized oxygen atom has been used to describe the properties of acetals (with two sp3 orbitals occupied each by two electrons pairs: rabbit ears). Nevertheless, electron distribution about oxygen atom in H2O, ethers and related compounds are better represented by using a sp2-hybridized oxygen atom. In sp2 hybridized oxygen atom, the sp2(O) orbital is a sub-HOMO equivalent to the combination 1/˚2 sp3(O) + 1/˚2 sp3(O) and the HOMO (localized on the ethereal oxygen atom) is equivalent to 1/˚2 sp3(O) – 1/˚2 sp3(O). As the latter is higher in energy (lower ionization energy) than the former localized orbital (higher ionization energy), it contributes more efficiently to any electron transfer, for instance into a hyper-conjugating C–X bond. Consequently, we prefer to use sp2-hybridized rather than sp3-hybridized oxygen atoms to describe the non bonding electron pairs involved in conformational anomeric effect. The σ conjugation of the type [MATHEMATICAL EXPRESSION NOT REPRODUCIBLE IN ASCII] (negative hyperconjugation) also intervene on the stability difference of 1,7-dioxaspiro[5.5]undecanes (6,6-spiroketals) (Fig. 11). In these systems, the contrasteric effect renders the more sterically encumbered gauche/gauche conformers (19) more stable than their anti/gauche (20) and anti/anti (21) conformers. Noteworthy, the model depicted in Fig. 11 for the conformational anomeric effect which intends to overlap between 2p(O) (HOMO) and σ*C–O (LUMO) orbitals is in agreement with the dihedral (torsional) angle y measured in 6,6-spiroketals. In fact, the analysis of the crystal structures of [6,6]-spiroketal derivatives recorded in the Cambridge Structural Database revealed that the dihedral angle y (Fig. 7) is larger than 60° in most cases {mean value: 62° for 84 [6,6]-(gauche/gauche)- spiroketal structures}.
3.3 Conformational anomeric effects in pyranosides
In the case of 2-hydroxytetrahydropyran, the axial conformer 22 is calculated to be more stable than its equatorial conformer 23 in vacuum (Fig. 12). Solvent effects change the equilibrium constant and the equatorial form 23 is favored in aqueous solution, in agreement with data. The magnitude of the conformational endo-anomeric effect in 22 is estimated to 2.0 kcal/mol (gas phase; stereoelectronic effects overwhelming the steric gauche effect, as given by the comparison of the free enthalpy difference between axial/equatorial 2-hydroxytetrahydropyran and cyclohexanol).
The hydroxyl group in 22 and 23 exhibits conformational exo-anomeric effect of 2.3 (22s [??] 22a) and 2.9 (23s [??] 23a) kcal/mol. Similar computational and experimental effects are found for 2-methoxytetra-hydropyrans (13 [??] 14).
(Continues...)
Excerpted from Carbohydrate Chemistry Volume 35 by Amelia Pilar Rauter, Thisbe K. Lindhorst. Copyright © 2009 The Royal Society of Chemistry. Excerpted by permission of The Royal Society of Chemistry.
All rights reserved. No part of this excerpt may be reproduced or reprinted without permission in writing from the publisher.
Excerpts are provided by Dial-A-Book Inc. solely for the personal use of visitors to this web site.
Table of Contents
Chapter 1: Natural Products as Drugs and Leads to Drugs: the Historical Perspective; Chapter 2: Chemical Space and the Difference between Natural Products and Synthetics; Chapter 3: The Importance of Mechanism of Action Studies; Chapter 4: The Convention on Biological Diversity and its Impact on Natural Product Research; Chapter 5: Plants: Revamping the Oldest Source of Medicines with Modern Science; Chapter 6: Macromarines: a Selective Account on the Potential of Marine Sponges, Molluscs, Soft Corals and Tunicates as a Source of Therapeutically Important Molecular Structures; Chapter 7: Microorganisms: their Role in the Discovery and Development of Medicines; Chapter 8: Advances in Biological Screening for Lead Discovery; Chapter 9: Advances in Instrumentation and Automation, Dereplication and Prefractionation; Chapter 10: Natural Product Combinatorial Biosynthesis: Promises and Realities; Chapter 11: A Snapshot of Natural Product-Derived Compounds in Late Stage Clinical Development at the End of 2008; Chapter 12: From Natural Product to Clinical Trials: NPI-0052 (Salinosporamide A), a Marine Actinomycete-Derived Anticancer Agent; Chapter 13: From Natural Product to Clinical Trials: Bevirimat, a Plant-Derived Anti-AIDS Drug; Chapter 14: Daptomycin; Chapter 15: Micafungin; Chapter 16: From rapamycin to temsirolimus;What People are Saying About This
"This volume serves as a useful reminder that even as the first decade of the 21st century comes to a close, natural products are still a valuable resource for the new therapeutic agents and new drug leads with broad structural diversity." "This is a timely and highly informative text that belongs in institutional libraries where biomedical research is carried out...many natural product researchers, as well as medicinal chemists, synthetic organic chemists, and pharmacologists will find this volume well worthy of purchase for their personal libraries."