Analog Optical Links: Theory and Practice available in Paperback
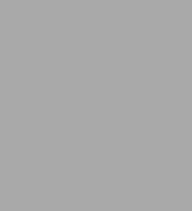
Analog Optical Links: Theory and Practice
- ISBN-10:
- 0521027780
- ISBN-13:
- 9780521027786
- Pub. Date:
- 11/02/2006
- Publisher:
- Cambridge University Press
- ISBN-10:
- 0521027780
- ISBN-13:
- 9780521027786
- Pub. Date:
- 11/02/2006
- Publisher:
- Cambridge University Press
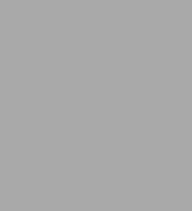
Analog Optical Links: Theory and Practice
Paperback
Buy New
$58.99Buy Used
$41.31-
SHIP THIS ITEMIn stock. Ships in 1-2 days.PICK UP IN STORE
Your local store may have stock of this item.
Available within 2 business hours
-
SHIP THIS ITEM
Temporarily Out of Stock Online
Please check back later for updated availability.
Overview
Product Details
ISBN-13: | 9780521027786 |
---|---|
Publisher: | Cambridge University Press |
Publication date: | 11/02/2006 |
Edition description: | Reissue |
Pages: | 304 |
Product dimensions: | 6.65(w) x 9.57(h) x 0.63(d) |
About the Author
Read an Excerpt
Cambridge University Press
0521621631 - Analog Optical Links - Theory and Practice - by Charles H. Cox, III
Excerpt
1
Introduction
1.1 Background
Optical communication links have probably been around for more than a millennium and have been under serious technical investigation for over a century, ever since Alexander Graham Bell experimented with them in the late 1800s. However, within the last decade or so optical links have moved into the communications mainstream with the availability of low loss optical fibers. There are of course many reasons for this, but from a link design point of view, the reason for fiber's popularity is that it provides a highly efficient and flexible means for coupling the optical source to a usually distant optical detector. For example, the optical loss of a typical terrestrial 10-km free-space optical link would be at least 41 dB1 (Gowar, 1983), whereas the loss of 10 km of optical fiber is about 3 dB at wavelengths of ~1.55 μm. To put the incredible clarity of optical fibers in perspective, if we take 0.3 dB∕km as a representative loss for present optical fibers, we see that they are more transparent than clear air, which at this wavelength has an attenuation of 0.4 to 1 dB∕km (Taylor and Yates, 1957).
Today the vast majority of fiber optic links are digital, for telecommunications and data networks. However, there is a growing, some might say exploding, number of applications for analog fiber optic links. In this case, the comparison is not between an optical fiber and free space but between an optical fiber and an electrical cable. Figure 1.1 shows typical cable and optical fiber losses vs. length. As can be seen, the highest loss for optical fiber is lower than even large coax for any usable frequency.
For the purposes of discussion in this book, an optical link will be defined as consisting of all the components required to convey an electrical signal over an optical carrier. As shown in Fig. 1.2, the most common form of an optical link can
Figure 1.1 Loss versus length for representative types of electrical cables and optical fibers at three common wavelengths.
Figure 1.2 Basic components of a fiber optic link: modulation device, optical fiber and photodetection device.
be implemented with just three principal parts. At the input end is a modulation device, which impresses the electrical signal onto the optical carrier. An optical fiber couples the modulation device output to the input of the photodetection device, which recovers the electrical signal from the optical carrier.
To make the link and some of the technical issues surrounding it more concrete, consider the following example. For the modulation device we will use a diode laser and for the photodetection device a photodiode. Both of these devices will be described in detail in Chapter 2, so for now it is sufficient to know that the former converts an electrical current into a corresponding optical intensity while the latter does the reverse - it converts an optical intensity into an electrical current. We will connect these two devices optically via a length of optical fiber.
Now let us send an RF signal over this simple link. When we measure the RF signal power that we recover from the photodiode we find that we typically only get 0.1% of the RF power we used to modulate the diode laser - i.e. an RF loss of 30 dB! This raises a host of questions, among them: where did the remaining 99.9% of the RF power go; do we always have to suffer this incredible loss; what
Figure 1.3 Typical loss vs. length of coax and optical fiber links operating at 10 GHz.
are the tradeoffs if we try to reduce this loss; how does this loss impact other link parameters such as the noise and distortion performance? It is the goal of this book to provide the background to answer such questions.
We can get an indication of the basis for these losses if we look at the typical loss of a link versus the length of the optical fiber between the modulation and photodetection devices. An example of this is shown in Fig. 1.3, which plots the RF loss vs. length for fiber and coaxial links operating at 10 GHz. The range of losses shown for the optical fiber link is representative of what has been reported to date. We can see that the fiber link loss increases slowly with fiber length as we would expect from the fiber optical loss data of Fig. 1.1, whereas the coax link loss increases much more quickly with length. However, note that at zero link length, the coaxial cable loss goes to zero while the optical fiber link loss does not. The zero length loss for the optical fiber link represents the combined effects of the RF/optical conversion inefficiencies of the modulation and photodetection devices. For long length links this zero length conversion loss is less important because the sum of the conversion and fiber losses is still less than the coaxial loss. But for shorter length links, where the fiber loss is negligible, the conversion loss dominates the link loss and exceeds the coaxial loss. Consequently an important aspect of link design will be understanding the reasons behind the conversion loss and developing techniques for reducing it.
In comparing an optical link with the coax or waveguide that it often replaces, there are a couple of important facts that impact link design, in addition to the loss vs. length issue we just discussed. One fact is that while the fiber is just as bi-directional as coax, when one includes the modulation and photodetection devices, the fiber link is uni-directional.2 (This is also true of coax, when one includes the driver and receiver electronics.) However, unlike the coax case, in the fiber link case the reverse transmission - i.e. from photodetection to modulation device - is truly zero. This is because the common modulation device has no photodetection capability and the typical photodetection device cannot produce optical emissions.3 The impacts of these facts for the link designer are that: (1) the application as well as the RF performance are part of the link design process and (2) the modulation and photodetection circuits are separable in that changing the loading at the photodetection device has no impact on the modulation device circuit.
Another distinction between an optical link and its RF counterpart is in the number of parameters needed to describe their use in a system. Coax and waveguide are completely defined for these purposes in terms of two parameters: their loss and frequency response. An optical link, which is more analogous to an active RF component - such as an amplifier - than to passive coax, typically requires at least four parameters: loss, bandwidth, noise figure and dynamic range. In terms of these four parameters, we will see that the modulation device has the greatest impact on all four parameters, with the photodetector a close second in terms of these same parameters. The fiber, especially when longer lengths are involved, can have a significant impact on loss, which as we will see in turn affects noise figure. The fiber can also indirectly affect bandwidth via its dispersion; fiber effects on dynamic range are negligible.
The emphasis in this book will be on developing the tools and techniques that will enable one to design links for a variety of applications, based on given device designs. This is quite different from other books where the emphasis is on designing devices, with secondary - at best - consideration on applying the device in a link. While the link models will be firmly rooted in the device physics, the focus here will be on relating device, and to a lesser extent fiber, parameters to link parameters.
Conceptually the RF signal could be conveyed over an optical link using any one of the optical carrier's parameters that are analogous to the parameters commonly used with an RF carrier: i.e. the optical carrier's amplitude Eo, frequency ν, or phase θ. For specificity, assume an optical plane wave propagating in free space in the z-direction:
Means exist in the optical domain that duplicate many of the functions in the RF domain: frequency mixing, LO generation, heterodyne conversion, optical amplifiers - one notable exception is the present lack of any method for hard optical limiting, as there is in the electronic domain. Indeed, optical modulators for each of the three parameters listed above have been demonstrated. However, the technology for optical receivers is at present roughly where RF receivers were at the beginning of the twentieth century.
Virtually all present RF receivers are coherent receivers in which the amplitude or frequency - or in some cases the phase - of the incoming carrier is detected. This is in contrast to the early days of radio when direct detection was the norm - i.e. detection of the presence/absence of the RF carrier without regard to its precise frequency and certainly without any phase information (e.g. Morse code).
Direct detection of an intensity modulated optical carrier is straightforward; as we will see in Chapter 2 all that is required is a photodiode (Yu, 1996). Demodulation of an optical carrier, which has been modulated in either frequency or phase, requires a coherent optical receiver. In turn this requires an optical local oscillator, optical mixer - which can be done in the photodiode - and optical filter. Although coherent optical receivers have been extensively studied (see for example Seeds, 1996; Yamamoto and Kimura, 1981) they have not found widespread application at present, primarily due to the fact that their marginal performance improvement over direct detection does not justify their significant additional complexity.
The results of the coherent optical receiver studies indicated that coherent detection offers greater sensitivity than direct detection. Although coherent detection links require about the same total optical power at the photodetector, they require less modulated optical power than direct detection for the same signal-to-noise ratio, when used in conjunction with a high optical power local oscillator. This fact was the driving force behind much of the early work on coherent detection. However, more recently, the availability of optical amplifiers, which can be used as optical pre-amplifiers before the photodetector, has permitted direct detection sensitivity to approach that of coherent detection.4
Although direct detection is much simpler to implement than coherent detection, it detects only the intensity of the optical wave; all frequency and phase information of the optical carrier is lost. We can see this by examining the intensity of the plane wave example from above. The intensity I (W∕m2) is
or simply the square of the optical wave's amplitude - when the amplitude is real - and where ɛ0 is the permittivity and c is the speed of light, both in vacuum. Consequently, amplitude and intensity modulation are not synonymous.5 One important aspect of this distinction is that the spectrum of the optical waveform for intensity modulation can be much wider than the RF spectrum of the modulating waveform, because the optical spectrum contains harmonics of the modulation waveform generated in the square-law modulation process. This situation is shown diagrammatically in Fig. 1.4(b) by the ellipses that indicate continuation of the sidebands on both sides of the optical carrier. For low modulation indices these harmonics may be negligible, in which case the intensity and amplitude modulated spectra have approximately the same bandwidth. The similarities that intensity and amplitude modulation do share often lead to these terms being used interchangeably in casual discussions. This is unfortunate because the unsupported assumption of equivalence can lead to erroneous conclusions.
Thus intensity modulation of the optical carrier followed by direct detection - which is often abbreviated IMDD - is the universal choice in applications today and will be the focus of this book.
There are two broad categories of optical intensity modulation (Cox et al., 1997). In the simple link example given above, and as shown in Fig. 1.4(a), with direct modulation, the electrical modulating signal is applied directly to the laser to change its output optical intensity. This implies that the modulating signal must be within the modulation bandwidth of the laser. As we will see, only semiconductor diode lasers have sufficient bandwidth to be of practical interest for direct modulation. The alternative to direct modulation is external, or indirect, modulation; see Fig. 1.4(c). With external modulation, the laser operates at a constant optical power (i.e. CW) and intensity modulation is impressed via a device that is typically external to the laser. Since there is no modulation requirement on the laser for external modulation, this removes a major restriction on the choice of lasers that can be used for external modulation. Both methods achieve the same end result - an intensity modulated optical carrier - and consequently both use the same detection method, a simple photodetector. As we will discuss in the chapters to follow, there are a number of fundamental and implementation issues concerning these two approaches that give each distinct advantages.
Ideally the electro-optic and opto-electronic conversions at the modulation and photodetection devices, respectively, would be highly efficient, strictly linear and
Figure 1.4 Intensity modulation, direct detection links (b) using (a) direct and (c) external modulation.
introduce no noise. Further the devices would maintain these characteristics over all frequencies and for any RF power no matter how large or small.
From the simple link example presented above, we saw that some practical electro-optic devices fall well short of the ideal conversion efficiency goal. As we will see in later chapters, practical devices often also fall short of the other ideal characteristics as well. For example, without proper design practices, we commonly find unacceptable levels of distortion in analog links - which means that one or more of the conversion processes is not strictly linear. Casual link design can also lead to additional noise being added by the optical link, which can reduce the link's ability to convey low level signals. At the other end of the RF power range, practical devices are also limited in the maximum RF power they can handle; typically above a certain RF power, there is no further increase in modulation and the device is said to have saturated.
As we go through this book we will develop the basis for the present limitations of practical devices, then present techniques for reducing these limitations. Our task as a link designer is complicated by the fact that reducing one parameter, such as the noise, often leads to an increase in another parameter, such as the distortion. The "art" of link design is finding one link design that balances the competing effect of several parameters.
1.2 Applications overview
There are, of course, many ways to review the current applications of analog optical links. The wide range of application requirements and frequency ranges makes it difficult to have a general comparative discussion of the links used in all such applications. One way to organize an introductory discussion is to recognize that the different application categories emphasize different technical requirements depending on the primary function the link is fulfilling. Consequently we can group fiber optic links into three functional categories that dominate the applications at present: transmit, distribution and receive links.
1.2.1 Transmit optical links
An optical link for transmit applications is aimed at conveying an RF signal from the signal source to an antenna, as shown in generic block diagram form in Fig. 1.5. Applications include the up-link for cellular/PCS antenna remoting and the transmit function of a radar system. Both direct and external modulation have been investigated for radar transmit applications whereas only direct modulation is presently used for cellular/PCS transmit applications.
Since high level signals are involved in transmit, noise is not usually a driving requirement. In radar applications, generally the link needs to convey only a single frequency at a time; consequently distortion is also not a driving requirement. However, in multi-function antennas and cellular/PCS up-links, multiple signals are present simultaneously, so there is the need to meet a distortion requirement, albeit a relatively modest one in comparison to receive applications.
Virtually all transmit applications do require a relatively high level RF signal to drive the antenna. As suggested by Fig. 1.3, most fiber optic links have significant RF-to- RF loss, and it turns out that this high loss also occurs at low frequencies such as UHF. In addition, the maximum RF power at the photodetector end of the link is typically limited by thermal and linearity constraints to about -10 dBm.6 Consequently for a transmit antenna to radiate 1 W means that 40 dB of RF gain is needed between the link output and the antenna. Further, if this link has a gain of -30 dB, then 20 dBm of input power is necesssary to produce -10 dBm at the link output. However, 20 dBm is above the saturation power of many modulation
Figure 1.5 Example block diagram of a fiber optic link used for true-time-delay beam steering in a phased array antenna.
devices, which means that a lower drive to the link and consequently a higher gain power amplifier after the link photodetector is typically required. Therefore there is a real need to decrease the RF/optical conversion loss and increase the output power capability of links for transmit applications.
The center frequency of the signal sent to the antenna can be anywhere from 10 MHz to 100 GHz. Complete links have been demonstrated up to 20 GHz. Consequently at center frequencies in this range, the transmit link is typically designed to convey the center frequency without any frequency translation.
The components necessary for higher frequency links have been demonstrated: broadband modulation of a diode laser up to 33 GHz (Ralston et al., 1994), of an external modulator up 70 GHz (Noguchi et al., 1994) and of a photodetector up to 500 GHz (Chou and Liu, 1992) has been reported. However, the efficiencies of these components are such that if they were combined into a link, the link gain without any amplifiers would be rather low. For instance, if the 70 GHz modulator were used in a link with the 500 GHz photodetector with 1 mW of incident optical power, the gain would be approximately -60 dB at 70 GHz. This level of performance only serves to extend the needs mentioned above to include reduced loss at high frequency as well.
1.2.2 Distribution optical links
This type of link is intended to distribute the same RF signal to a multiplicity of sites, such as distributing the phase reference within a phased array radar. The first large scale commercial application of analog fiber optic links was the distribution of cable television (CATV) signals (see for example Darcie and Bodeep, 1990; Olshansky et al., 1989). As shown in Fig. 1.6, the low loss of optical fibers permitted reducing or even eliminating the myriad repeater amplifiers that had been required with coaxial distribution. Like the transmit links, distribution links convey relatively high level
Figure 1.6 Conceptual block diagram of optical-fiber-based CATV distribution system.
signals, consequently link noise is not a driving parameter. Also like some transmit links, distribution links that broadcast the phase reference, such as in a radar, have only a single frequency present at any one time, therefore distortion is not a driving parameter. However, in other distribution applications, such as CATV, multiple carriers - which can be as many as 80 in current CATV systems - are present simultaneously, so distortion becomes a key link parameter. Further, for CATV distribution, the bandwidth is sufficiently wide that both narrow-band and wide-band distortion - two terms that will be defined in Chapter 6 - must be taken into consideration in designing links for this application. Typically, external modulation links are used in the primary ring and direct modulation is used in the secondary ring.
Distribution links by their very nature have a high optical loss that is dominated by the splitting loss in the distribution network, which arises from dividing the optical signal among multiple photodetectors. For example, a fiber network that needs to distribute a signal to 100 locations would have an optical splitting loss of 20 dB, assuming ideal optical splitters that introduce no excess loss in the splitting process. As we will see in Chapter 3, 20 dB of optical loss translates to 40 dB of RF loss between the RF input and any one of the RF outputs. Although the total modulated optical power required is high, the power on each individual photodetector is low.
One convenient way to overcome the high splitting loss is by the use of optical amplifiers. The two basic types of optical amplifiers are semiconductor (see for example O'Mahony, 7 (see for example Desurvire, 1994), which although available at either wavelength are at present only commercially viable at the longer wavelength fiber band of 1.55 μm in the form of erbium-doped fibers. Either type of amplifier is capable of 30 to 40 dB of optical gain, but since these amplifiers have equal gain in either direction, optical reflections in links using them must be minimized to avoid spurious lasing.
© Cambridge University Press