Electron Microprobe Analysis and Scanning Electron Microscopy in Geology / Edition 2 available in Paperback
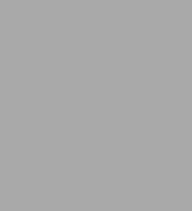
Electron Microprobe Analysis and Scanning Electron Microscopy in Geology / Edition 2
- ISBN-10:
- 052114230X
- ISBN-13:
- 9780521142304
- Pub. Date:
- 06/10/2010
- Publisher:
- Cambridge University Press
- ISBN-10:
- 052114230X
- ISBN-13:
- 9780521142304
- Pub. Date:
- 06/10/2010
- Publisher:
- Cambridge University Press
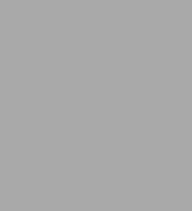
Electron Microprobe Analysis and Scanning Electron Microscopy in Geology / Edition 2
Paperback
Buy New
$59.99Buy Used
$32.00-
SHIP THIS ITEMIn stock. Ships in 1-2 days.PICK UP IN STORE
Your local store may have stock of this item.
Available within 2 business hours
-
SHIP THIS ITEM
Temporarily Out of Stock Online
Please check back later for updated availability.
Overview
Product Details
ISBN-13: | 9780521142304 |
---|---|
Publisher: | Cambridge University Press |
Publication date: | 06/10/2010 |
Edition description: | Second Edition |
Pages: | 212 |
Product dimensions: | 6.70(w) x 9.60(h) x 0.50(d) |
Read an Excerpt
Cambridge University Press
052184875X - Electron Microprobe Analysis and Scanning Electron Microscopy in Geology - by S. J. B. Reed
Excerpt
1
Introduction
1.1 Electron microprobe analysis
Electron microprobe analysis (EMPA) is a technique for chemically analysing small selected areas of solid samples, in which X-rays are excited by a focussed electron beam. (The term 'electron probe microanalysis', or EPMA, is synonymous.) The X-ray spectrum contains lines characteristic of the elements present; hence a qualitative analysis is easy to obtain by identifying the lines from their wavelengths (or photon energies). By comparing their intensities with those emitted from standard samples (pure elements or compounds of known composition) it is also possible to determine the concentrations of the elements quantitatively. Accuracy approaching ±1% (relative) is obtainable and detection limits down to tens of parts per million (by weight) can be attained. Under normal conditions, spatial resolution is limited to about 1 μm by the spreading of the beam within the sample. The spatial distributions of specific elements can be recorded in the form of line profiles or two-dimensional 'maps', which are commonly displayed using a 'false' colour scale to represent elemental concentrations.
1.2 Scanning electron microscopy
The scanning electron microscope (SEM) is a close relative of the electron microprobe (EMP) but is designed primarily for imaging rather than analysis. Images are produced by scanning the beam while displaying the signal from an electron detector on a TV screen or computer monitor. By choosing the appropriate detection mode, either topographic or compositional contrast can be obtained. ('Composition' here refers to mean atomic number: individual elements cannot be distinguished.) Spatial resolution better than 10 nm in topographic mode and 100 nm in compositional mode can be achieved, though in many applications the large depth of field in SEM images (typically at least 100 times greater than for a comparable optical microscope) is more relevant than high resolution. An important factor in the success of the SEM is that images of three-dimensional objects are usually amenable to immediate intuitive interpretation by the observer. The range of applications of SEM can be extended by adding other types of detector, e.g. for light emission caused by electron bombardment, or cathodoluminescence (CL).
1.2.1 Use of SEM for analysis
Scanning electron microscopes commonly have an X-ray spectrometer attached, enabling the characteristic X-rays of a selected element to be used to produce an image. Also, with a stationary beam, point analyses can be obtained, as in EMPA. (The spatial resolution with respect to analysis is, however, still limited to about 1 μm by beam spreading, despite the higher resolution obtainable in scanning images.) Since EMP instruments have electron imaging facilities, used primarily for locating points for analysis, the functions of the two instruments overlap considerably. The SEM is optimised for imaging, with analysis as an extra, whereas in the EMP the priorities are reversed and various additional features that facilitate analysis are incorporated.
1.3 Geological applications of SEM and EMPA
The advantages of the SEM as an imaging instrument (high spatial resolution, large depth of field, and simple specimen preparation) make it an invaluable tool in the following branches of geology.
Palaeontology. The SEM is ideally suited to the study of fossil morphology, especially that of micro-fossils.
Sedimentology. Three-dimensional images of individual sediment grains and intergrowths can be obtained; data on fabric and porosity can also be generated.
Mineralogy. The SEM is very effective for studying crystal morphology on a micro-scale.
Petrology. The ability to produce images of polished sections showing differences in mean atomic number is very useful both in sedimentary and in igneous petrology.
The reasons for the widespread application of EMPA to geology (whether carried out in a 'true' EMP instrument or SEM with X-ray spectrometer fitted), especially in the fields of mineralogy and petrology, can be summarised as follows.
(1) Specimen preparation is straightforward and entails the use of existing techniques of section-making and polishing with only minor modifications.
(2) The technique is non-destructive, unlike most other analytical techniques.
(3) Quantitative elemental analysis with accuracy in the region of ±1% (for major elements) can be obtained.
(4) All elements above atomic number 3 can be determined (with somewhat varying accuracy and sensitivity).
(5) Detection limits are low enough to enable minor and trace elements to be determined in many cases.
(6) The time per analysis is reasonably short (usually between 1 and 5 min).
(7) Spatial resolution of the order of 1 μm enables most features of interest to be resolved.
(8) Individual mineral grains can be analysed in situ, with their textural relationships undisturbed.
(9) A high specimen throughput rate is possible, the time required for changing specimens being quite short.
These characteristics have proved useful in the following subject areas.
Descriptive petrology. The EMPA technique is commonly used for the petrological description and classification of rocks and has an importance comparable to that of the polarising microscope.
Mineral identification. As an adjunct to polarised-light microscopy and X-ray diffraction, EMPA provides compositional information that assists in mineral identification.
Experimental petrology. For experimental studies on phase relationships and elemental partitioning between coexisting phases, the spatial resolution of the electron microprobe is especially useful, given the typically small grain size.
Geothermobarometry. The EMPA technique is ideally suited to the determination of the composition of coexisting phases in rocks, from which temperatures and pressures of formation can be derived.
Age determination. Th-U-Pb dating of minerals containing insignificant amounts of non-radiogenic Pb (such as monazite) is possible by EMPA, with higher spatial resolution than can be obtained with isotopic methods, though lower accuracy.
Zoning. The high spatial resolution of the technique enables zoning within mineral grains to be studied in detail.
Diffusion studies. Experimental diffusion profiles in geologically relevant systems can be determined with the electron microprobe, its high spatial resolution being crucial in this field.
Modal analysis. Volume fractions of minerals and other data can be obtained by automated modal analysis, mineral identification being based on X-ray and sometimes backscattered-electron signals.
Rare-phase location. Grains of rare phases can be located by automated search procedures, using the X-ray signal for one or more diagnostic elements.
1.4 Related techniques
Though EMPA has many useful attributes, as described in the previous section, other, complementary analytical methods offer advantages in one respect or another. These are outlined briefly in the following sections.
1.4.1 Analytical electron microscopy
With specimens less than 100 nm thick and an electron energy of at least 100 keV, much better spatial resolution (down to 10 nm) can be obtained, owing to the relatively small amount of lateral scattering which occurs as the electrons pass through the specimen. The reduction in X-ray intensity can be compensated to a large extent by using an X-ray detector of high collection efficiency and a high-intensity electron source. This type of analysis can be carried out in an 'analytical electron microscope' (AEM), in which conventional electron transmission imaging and diffraction capabilities are combined with X-ray detection. Analysis with high spatial resolution is also possible with a scanning transmission electron microscope (STEM) fitted with an X-ray spectrometer.
Another analytical technique that is also available in AEM and STEM instruments is electron energy-loss spectrometry ('EELS'), which utilises steps in the energy spectrum of transmitted electrons caused by energy losses associated with inner-shell ionisation. Electron spectrometers with parallel collection make this a very sensitive technique.
For further information about AEM, see Joy, Romig and Goldstein (1986) and Champness (1995).
1.4.2 Proton-induced X-ray emission
Characteristic X-rays can be excited by bombardment with protons, giving rise to the technique known as 'PIXE' (proton-induced X-ray emission). The principal advantage of PIXE is that the X-ray background is much lower than in EMPA (a consequence of the higher mass of the proton compared with the electron), making small peaks easier to detect. Detection limits are thus typically an order of magnitude lower (in the ppm range). On the other hand, high-energy protons are more difficult to focus in order to obtain high spatial resolution (a beam diameter of 1 μm is attainable, but only with low current), and they penetrate much further in solid materials. Protons of energy 1-4 MeV, which give efficient X-ray excitation, can penetrate the full 30-μm thickness of a petrological thin section and it follows that the spatial resolution with respect to depth is relatively poor. The equipment is quite costly and not very widely available, so geological applications have been fairly limited.
For more details about PIXE, see Fraser (1995), Halden, Campbell and Teesdale (1995) and Cabri and Campbell (1998).
1.4.3 X-ray fluorescence analysis
An alternative way of exciting characteristic X-rays is to bombard the specimen with X-rays of higher energy, this technique being known as X-ray fluorescence (XRF) analysis. It has been a standard method of elemental analysis in geology for a long time and offers good accuracy for major elements and detection limits in the region of 1 ppm. In its usual form it is a bulk method, requiring a significant amount of sample for analysis, and is therefore used principally for analysing whole rocks or separated minerals. An electron microprobe or SEM can, however, be converted to make it capable of XRF analysis with a spatial resolution of about 100 μm, by using the beam to excite X-rays in a target close to the specimen, in which fluorescent X-rays are excited.
The advent of synchrotron X-ray sources has revolutionised the possibilities of XRF analysis. The extremely high X-ray intensities available from these sources enable intense beams down to 1 μm in diameter to be produced for exciting the specimen, giving a microprobe technique in which high spatial resolution and low detection limits are combined. The accessibility of this technique is restricted by the limited number of synchrotrons in existence. For more information, see Smith and Rivers (1995). Relatively compact XRF analysers with high spatial resolution, using a low-power X-ray tube with a focussing device, are also available.
1.4.4 Auger analysis
The process known as the 'Auger effect', whereby an atom excited by electron bombardment may dissipate its energy by ejecting an electron rather than by characteristic X-ray emission, gives rise to an alternative method of analysis, which exploits the fact that the electron spectrum contains lines that have energies related to the atomic energy levels and are therefore characteristic of the element. Auger analysis is most effective for elements of atomic number below 10, for which X-ray analysis is least sensitive. Also, it differs in being a surface analysis technique, only electrons originating from depths of the order of 10 nm being detected. The scanning Auger microscope (SAM) is a close relative of the SEM, but is orientated towards the requirements of the electron energy analyser, and the instrument has to be operated at ultra-high vacuum.
This technique and its applications in geology are discussed in Section 4.8.6.
1.4.5 Ion microprobe analysis
Ion microprobe analysis is complementary to EMPA in its capabilities but has a completely different physical basis. This technique is a form of secondary-ion mass spectrometry (SIMS), whereby the specimen is bombarded with 'primary' ions, and ionised atoms from the sample ('secondary' ions) are collected and passed through a mass spectrometer. In the ion microprobe the primary ions are focussed to form a beam typically a few micrometres in diameter. For elemental analysis the advantages of the technique are low detection limits (much lower for some elements than others) and the ability to detect light elements (including H and Li) with high sensitivity. For many elements the ion microprobe lowers detection limits by several orders of magnitude compared with EMPA. Its value is enhanced by the possibility of obtaining isotopic data on small areas.
For further details, see Hinton (1995) and McMahon and Cabri (1998).
1.4.6 Laser microprobe methods
Another means by which micro-volumes of sample can be removed for analysis is bombardment with a focussed laser beam. This can be used in various ways. For example, in laser-induced mass spectrometry (LIMS) ions are generated directly by the laser beam and subjected to mass spectrometry. An alternative approach is to use less intense bombardment to generate local heating and release gases such as Ar, which can be transferred to a mass spectrometer, enabling isotope data to be obtained from selected areas. Yet another technique is to remove (or 'ablate') material and transfer this to an 'inductively coupled plasma' (ICP) source, from which either ions for mass spectrometry or light for optical emission spectrometry can be produced. The spatial resolution of these techniques is typically a few micrometres.
For further details of these techniques, see Perkins and Pearce (1995).
2
Electron-specimen interactions
2.1 Introduction
Electrons impinging on solid materials are slowed down principally through 'inelastic' interactions with outer atomic electrons, while 'elastic' deflections by atomic nuclei determine their spatial distribution. Some leave the target again, having been deflected through an angle of more than 90°. Both these 'backscattered' electrons and 'secondary' electrons dislodged from the surface of the sample are used for image formation. In addition, interactions between bombarding electrons and atomic nuclei give rise to the emission of X-ray photons with any energy up to E0, the energy of the incident electrons, resulting in a 'continuous X-ray spectrum' (or 'continuum'). 'Characteristic' X-rays (used for chemical analysis) are produced by electron transitions between inner atomic energy levels, following the creation of a vacancy by the ejection of an inner-shell electron.
2.2 Inelastic scattering
In the SEM or EMP the electrons with which the specimen is bombarded have an energy typically in the range 5-30 keV*, which is dissipated in interactions of various types with bound electrons and the lattice, known collectively as 'inelastic scattering'. Individual energy losses are mostly small; hence it is a reasonable approximation to assume that the electron decelerates smoothly as a function of distance travelled. The rate of energy loss is dependent on the property of the target material known as the 'stopping power', defined as -dE ⁄ d(ρs), where ρ is the density of the target and s the distance travelled.
Image not available in HTML version |
Fig. 2.1. Electron range (defined as the mean straight-line distance from start to finish of electron trajectory in the target) as a function of incident electron energy (E0) for target elements of various atomic numbers, calculated from the expression of Kanaya and Okayama (1972).
2.2.1 Electron range
The path length of electrons of a given initial energy is inversely proportional to density, and the product of distance travelled and density ('mass penetration') is approximately constant for all elements. The 'range', r, defined as the straight-line distance between the point of entry of an electron and its final resting place, is related to the path length, but is also influenced by elastic scattering (see Section 2.3), which causes the electrons to follow zig-zag paths. The following expression (Kanaya and Okayama, 1972) is useful for obtaining an estimate of r (in micrometres):
Display matter not available in HTML version |
Ranges for various elements, as a function of E0, are plotted in Fig. 2.1.
2.3 Elastic scattering
Elastic interactions with atomic nuclei involve large deflections in which there is little transfer of energy, owing to the large mass of the nucleus compared with that of the electron (Fig. 2.2). The angular deflection γ, as derived by Rutherford from classical mechanics, is given by
Image not available in HTML version |
Fig. 2.2. Elastic scattering: an incident electron is deflected (without significant energy loss) by the attractive force experienced in passing close to a positively charged nucleus.
Display matter not available in HTML version |
where p is the minimum distance (in nanometres) between the undeflected electron path and the nucleus, and is known as the 'impact parameter'. It follows from Eq. (2.2) that elastic scattering is greatest for heavy elements and low electron energies.
Computer simulations of electron trajectories are useful for modelling the spatial distribution of electrons in the target. For this purpose the 'Monte Carlo' method is used: this entails dividing the electron path into short sections and using random numbers to replicate the role of chance in determining the deflection angle (Joy, 1995). Figure 2.3 shows an example of the result of such a simulation.
2.3.1 Backscattering
There is a finite probability of an incident electron being deflected through an angle greater than 90o and emerging from the surface of the target. A similar result can also be obtained as a result of multiple deflections through smaller angles. The fraction of incident electrons which leave the specimen in this way is known as the backscattering coefficient (η) and is strongly dependent on atomic number, because of the increasing probability of high-angle deflection with increasing Z (see Eq. (2.2)). The relationship between η and Z takes the form shown in Fig. 2.4 for normal-incidence electrons (η is greater for oblique angles of incidence). Backscattered electrons have energies ranging up to E0 (the incident electron energy), and the mean of the energy distribution is highest for elements of high atomic number, for which there is a relatively large probability of high-angle deflections, by comparison with low-Z elements for which multiple low-angle scattering dominates and more energy is lost before the electrons emerge.
Image not available in HTML version |
Fig. 2.3. Computer simulations by the Monte Carlo method: (a) electron trajectories and (b) X-ray emission (each dot represents the emission of a photon), for 20-keV incident electrons and a silicon target (side of rectangle = 3μm). (By courtesy of P. Duncumb.)
Image not available in HTML version |
Fig. 2.4. Backscattering coefficient (η) versus atomic number; η is the fraction of incident electrons that leave the target and is almost independent of incident electron energy over the normal range.
© Cambridge University Press