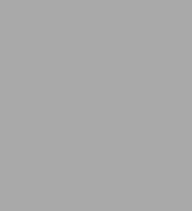
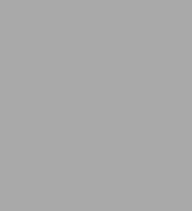
eBook
Related collections and offers
Overview
The author considers the first and second laws of thermodynamics in turn, after which he proceeds to applications of thermodynamic principles. He devotes considerable attention to the concept of entropy, emphasizing the interpretation of entropy changes and chemical behavior in terms of qualitative molecular properties. Students gain a familiarity with the entropy concept that will form a solid foundation for later courses and more formal thermodynamic treatments.
Product Details
ISBN-13: | 9780486151236 |
---|---|
Publisher: | Dover Publications |
Publication date: | 02/13/2013 |
Series: | Dover Books on Chemistry |
Sold by: | Barnes & Noble |
Format: | eBook |
Pages: | 176 |
File size: | 8 MB |
Read an Excerpt
Elementary Chemical Thermodynamics
By Bruce H. Mahan
Dover Publications, Inc.
Copyright © 2006 Dover Publications, Inc.All rights reserved.
ISBN: 978-0-486-15123-6
CHAPTER 1
Introduction
THERMODYNAMICS provides the most general and efficient methods for studying and understanding complex physical phenomena, and thus it should be one of the first subjects studied by a student who has a serious interest in chemistry or any physical science. The features of the physical world that are most obvious to us are not the atomic properties, which we detect only with sophisticated devices, but rather the gross properties of matter obvious to one of our five senses or measurable with simple apparatus. These are properties such as pressure, volume, temperature, and composition—properties of matter in bulk, rather than of individual isolated mol
The properties of matter which are so obvious to us are called macroscopic properties, and they are, naturally, the first features we use to describe a physical situation. Thermodynamics deals only with these macroscopic quantities; the concept of a molecule need never be used in a thermodynamic argument.
To the student of elementary chemistry trained to speak so casually of atoms and molecules, the absence of the atomic concept from thermodynamics may seem to be a serious weakness. The opposite is true. The great strength of thermodynamics is that it makes no use of the subtle and sometimes flimsy theories of molecular structure. The only quantities and concepts which enter thermodynamics are the experimentally measurable macroscopic properties of matter. Thermodynamics provides a framework for systematizing, discussing, and relating these properties. That is one of the reasons why the subject is so important to the understanding of the physical world and why it deserves careful study by the beginning student.
Thermodynamics does more than provide a useful scientific language; it calls attention to the two macroscopic properties which are most fundamentally responsible for the behavior of matter. The first of these quantities is energy; the second, less familiar, is entropy. We say that these quantities are most fundamental because the eventual course of all physical events can be summarized by two statements:
The energy of the universe is conserved.
The entropy of the universe increases.
These are the first and second laws of thermodynamics. An understanding of these statements, and of how energy and entropy depend on the other physical properties of matter, allows us to establish criteria for predicting the extent to which a chemical reaction may proceed under a given set of conditions. That is, thermodynamics can show us how to calculate the equilibrium constant of a reaction which has never been run, just by using data obtained from measurements of the individual properties of the pure reactants and products. Furthermore, it can tell us how that equilibrium constant will vary as temperature is changed. We shall find that there is a wealth of other applications in which thermodynamic analysis is used to obtain a maximum amount of information from experiments that are conveniently done; thereby, experiments that are difficult, or practically impossible, are avoided.
Naturally, an examination of only the macroscopic properties of matter does not completely satisfy anyone familiar with the concepts and theories of molecular structure and behavior. A working scientist always tries to explain his observations of macroscopic properties in terms of what he knows of molecular structure. While thermodynamics is not a molecular theory, it does simplify the job of understanding physical phenomena in terms of molecular properties. Thermodynamics shows clearly that energy and entropy are the quantities that control the behavior of matter. Thus, theories of molecular structure have a well-defined question to answer: How do energy and entropy depend on the structure and properties of individual molecules? In what follows we shall demonstrate the use of thermodynamic concepts in solving chemical problems; but as opportunity allows, we shall also try to explain the qualitative connection between thermodynamics and molecular structure.
1-1 THERMODYNAMIC SYSTEMS
The most efficient way to learn about the behavior of matter is to conduct controlled experiments. Naturally, at any one time we can make measurements on only a small segment of the physical universe, and it is necessary to define the limits of this segment quite carefully. The part of the universe under investigation in an experiment is called the system, and all other objects which may act on the system are called the surroundings.
In the most fortunate instances, the boundaries which separate the system from its surroundings are experimentally well defined. For example, there is no question that for most reactions which occur in aqueous solution it is perfectly legitimate to claim that the glass beaker which contains the solution is not part of the reaction system, but instead forms a rather innocuous part of the surroundings. If we were concerned with the solubility of a salt in water, a few experiments with Pyrex, quartz, and porcelain containers would demonstrate that the concentration of a saturated solution is independent of the nature of the vessel in which it is contained. It would then be proper to claim that the solubility we had determined was a property of the system salt and water, and not of the system salt and water and beaker.
As another example of the importance of realizing the limits of a system, suppose we wish to investigate the relation between the pressure and volume of a certain amount of gas. If we do this experiment in a rigid steel cylinder equipped with a frictionless piston, then as we increase the pressure on the gas at a constant temperature, the change in volume is determined by the properties of the gas alone. In this case it is correct to say that the pressure-volume properties of the system are those of a gas.
On the other hand, if we had placed the same volume of gas in a rubber balloon and then varied the pressure on the balloon, the observed change in volume would be determined not only by the properties of the gas but also by the elastic properties of the balloon. In this case the system would have to be regarded as the gas and its container. Clearly, if we were interested only in the properties of the gas, we would choose to investigate it by using the steel cylinder and piston.
1-2 STATES AND STATE FUNCTIONS
After we have defined the limits of the system which we choose to discuss or investigate, we can proceed to describe the properties of the system. The purpose of this description is to allow any other scientist to reconstruct the system in all its important detail so that his replica will behave identically to the original. The description of the system therefore must be complete, but it is also desirable that it be as concise as possible. That is, it should be limited to information which actually affects the measured behavior of the system.
For example, if we have the simple system of a single particle of known mass acted upon by a known force such as gravity, experience tells us that it can be completely described by giving the values of its position and velocity. In other words, we have observed repeatedly that all single particles of mass m placed at a certain point x, y, z above the earth and given certain velocity components υx, υy, and υz will behave the same. Therefore, we conclude that the only information necessary to completely describe the condition of the particle are its positional coordinates and velocity components. All other properties of the single-particle system, such as its kinetic or potential energy, are fixed by these six numbers.
The act of completely describing a system is called specifying its state. As we have just seen, the state of a single-particle system can be specified by only six numbers. The systems which we shall study in thermodynamics are much more complicated, however. For example, a mole of gas is a typical thermodynamic system, and since six numbers are necessary to specify the state of a single particle, it would appear that 6 X 6 X 1023 numbers are required to specify the state of a mole of gas. Fortunately, this much information is not necessary. In fact, for the purposes of thermodynamics the state of a mole of gas can be completely specified by giving the values of only two of the macroscopic properties pressure, volume, and temperature.
The reason for this enormous simplification lies in the nature of the common laboratory experiments with which thermodynamics is concerned. In these experiments we use devices such as meter sticks, thermometers, and manometers, or their more sophisticated equivalents, to examine the properties of systems. None of these instruments is sensitive to the behavior of single atomic particles. None of them gives us a record of the position and velocity of each atom or molecule. Instead, each of these devices is sensitive to a general property produced by the average behavior of all the particles in the system. In effect, these instruments are too large to respond to the behavior of the individual submicroscopic particles. The properties to which they do respond are macroscopic properties of the system as a whole.
As long as we use measuring devices which are sensitive only to the macroscopic properties of matter, the detailed knowledge of the position and velocity of individual atoms in a system is not required in order to describe the conditions or results of our experiments. Thermodynamics is concerned only with the macroscopic properties of matter; therefore, the thermodynamic state of a system can be completely described in terms of a few macroscopic quantities.
The macroscopic quantities that are used to specify the state of a thermodynamic system are called state variables or state functions, because their values depend only on the condition, or state, of the thermodynamic system. There are algebraic relationships between the state variables of a thermodynamic system. For instance, for a given amount of material, pressure, volume, and temperature are not all independent of each other but are connected by a mathematical relation called the equation of state. The simplest example of such a relation is the ideal-gas equation of state, PV = nRT. Liquids, solids, and nonideal gases have equations of state which are generally considerably more complicated. The nature of the atoms in a system determines the algebraic form of the equation of state, and thus this equation is one of the properties which characterizes a system.
It is very important to understand the significance of the term "state function." We shall see presently that, in addition to pressure, volume, and temperature, energy is a state function; and there are many others. All state functions have the following important property: Once we specify the state of a system by giving the values of a few of the state functions, the values of all other state functions are fixed. We can use the ideal-gas equation of state to demonstrate this fact. When the pressure and temperature of one mole of an ideal gas are specified, the volume must assume the value V = RT/P. All other state functions such as energy also automatically assume definite values which are determined by the values of P and T.
State functions have one other important property. When the state of a system is altered, the change in any state function depends only on the initial and final states of the system, and not on how the change is accomplished. For example, when a gas is compressed from an initial pressure P1 to a final pressure P2, the change in pressure ΔP is given by the expression
ΔP = P2 - P1
Only the initial and final values of the pressure determine ΔP. Any intermediate values which P may have assumed in changing from P1 to P2 are immaterial.
This is by no means a trivial property, and it is possessed only by state functions. By way of analogy, we can say that any person who travels from Boston to San Francisco has changed his position (or state) on earth by an amount that depends only on the location of his initial state (Boston) and his final state (San Francisco). However, the distance he travels depends not only on the location of the cities but also on the path he takes from one to the other. Thus, position is a state function, but distance traveled is not. These are the significant properties of state functions, and it is these properties which, we shall find, make state functions so useful.
1-3 EQUILIBRIUM STATES
There are some conditions of thermodynamic systems which cannot be described in terms of state functions. For example, suppose our system is a gas confined in a cylinder with a movable piston. When the piston is motionless, as in Fig. 1–1a, the state of the gas can be specified by giving the values of its pressure and temperature. However, if the gas is suddenly compressed, as in Fig. 1–1b, its state cannot be described in terms of one pressure and temperature. While the piston is moving, the gas immediately in front of the piston is compressed and heated, whereas the gas at the far end of the cylinder is not. There is, then, no such thing as the pressure or temperature of the gas as a whole. Conditions in which the state variables are changing in time and space, called nonequilibrium states, are not treated by thermodynamics. Thermodynamics deals only with equilibrium states in which the state variables have values that are uniform and constant throughout the whole system.
Let us examine the criteria for an equilibrium state more carefully. First, the mechanical properties of a system must be uniform and constant. This means that there must be no unbalanced forces acting on or within a system, since any unbalanced forces would cause the volume to change continuously and we would be unable to specify the state of the system.
Second, the chemical composition of a system at equilibrium must be uniform, and there must be no net chemical reactions taking place. The occurrence of any net chemical change would inevitably change such properties of a system as its density or temperature and make specification of its state impossible.
The third and final criterion of an equilibrium state is that the temperature of the system must be uniform and must be the same as the temperature of the surroundings. Whenever a temperature difference exists, heat tends to flow until the temperature difference disappears. Any system in which heat is flowing has macroscopic properties which are not uniform and which may be changing in time; therefore, such a system cannot be in an equilibrium state.
1-4 TEMPERATURE
We. have emphasized that state functions are of foremost importance in thermodynamics. Some of these state functions like pressure, volume, and chemical composition, are familiar quantities and need no elaborate explanation. On the other hand, temperature, although a common quantity, has rather subtle conceptual origins. The idea of a quantitative temperature was introduced because it became obvious that the results of many experiments depended on what we qualitatively experience as "hotness."
The first step in creating a temperature scale is to find some convenient property of matter which depends in a simple way on hotness. Temperature can be indicated, for example, by the density of liquid mercury, which is commonly measured by the distance mercury expands from a bulb into a glass capillary tube. The centigrade temperature scale is defined by assigning a value of zero temperature units, or degrees, to the length of the mercury column when the thermometer is immersed in an ice-water bath and 100 degrees to the length of the column when the thermometer is in contact with water at its normal boiling point. Intermediate temperatures are defined by placing 99 equally spaced marks between the two calibration points. It is clear that the temperature indicated by this thermometer depends on the properties of the materials used in its construction. Furthermore, by dividing the length between 0° and 100° into 100 equal units, we are really saying that temperature is something which depends linearly on the volume of mercury.
If some other liquid is used in the thermometer, the resulting temperature scale is different. For example, suppose we use water as the working liquid. We mark the thermometer in the same way, noting the length of the water column at the ice point and boiling point and dividing the interval into 100 equal units. Now we put our mercury thermometer and water thermometer in a bath at 0° and slowly raise the temperature. When the mercury thermometer reads 4°, the water thermometer reads -0.36°. This happens because, as the temperature is raised from 0° to 4° on the mercury scale, water contracts instead of expanding. If we used the properties of water to define temperature, we would have to say that when hotness increases, temperature sometimes goes up and sometimes goes down.
(Continues...)
Excerpted from Elementary Chemical Thermodynamics by Bruce H. Mahan. Copyright © 2006 Dover Publications, Inc.. Excerpted by permission of Dover Publications, Inc..
All rights reserved. No part of this excerpt may be reproduced or reprinted without permission in writing from the publisher.
Excerpts are provided by Dial-A-Book Inc. solely for the personal use of visitors to this web site.
Table of Contents
I. IntroductionII. The First Law of Thermodynamics
III The Second Law of Thermodynamics
IV. Applications of the Thermodynamic Principles
Index