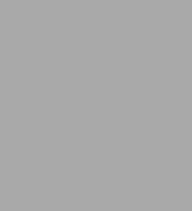
Our Renewable Future: Laying the Path for One Hundred Percent Clean Energy
216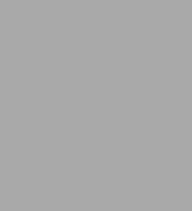
Our Renewable Future: Laying the Path for One Hundred Percent Clean Energy
216Overview
The next few decades will see a profound energy transformation throughout the world. By the end of the century (and perhaps sooner), we will shift from fossil fuel dependence to rely primarily on renewable sources like solar, wind, biomass, and geothermal power. Driven by the need to avert catastrophic climate change and by the depletion of easily accessible oil, coal, and natural gas, this transformation will entail a major shift in how we live. What might a 100% renewable future look like? Which technologies will play a crucial role in our energy future? What challenges will we face in this transition? And how can we make sure our new system is just and equitable?
In Our Renewable Future, energy expert Richard Heinberg and scientist David Fridley explore the challenges and opportunities presented by the shift to renewable energy. Beginning with a comprehensive overview of our current energy system, the authors survey issues of energy supply and demand in key sectors of the economy, including electricity generation, transportation, buildings, and manufacturing. In their detailed review of each sector, the authors examine the most crucial challenges we face, from intermittency in fuel sources to energy storage and grid redesign. The book concludes with a discussion of energy and equity and a summary of key lessons and steps forward at the individual, community, and national level.
The transition to clean energy will not be a simple matter of replacing coal with wind power or oil with solar; it will require us to adapt our energy usage as dramatically as we adapt our energy sources. Our Renewable Future is a clear-eyed and urgent guide to this transformation that will be a crucial resource for policymakers and energy activists.
Product Details
ISBN-13: | 9781610917803 |
---|---|
Publisher: | Island Press |
Publication date: | 06/02/2016 |
Sold by: | Barnes & Noble |
Format: | eBook |
Pages: | 216 |
File size: | 18 MB |
Note: | This product may take a few minutes to download. |
About the Author
David Fridley has been a staff scientist at the Energy Analysis Program at the Lawrence Berkeley National Laboratory in California since 1995. He is also Deputy Group Leader of Lawrence Berkeley’s China Energy Group, which collaborates with China on end-user energy efficiency, government energy management programs, and energy policy research. Fridley has written and spoken extensively on the energy and ecological limits of biofuels and serves as Renewable Energy & Energy Efficiency Fellow at Post Carbon Institute.
Read an Excerpt
Our Renewable Future
Laying the Path for 100% Clean Energy
By Richard Heinberg, David Fridley
ISLAND PRESS
Copyright © 2016 Post Carbon InstituteAll rights reserved.
ISBN: 978-1-61091-780-3
CHAPTER 1
Energy 101
It is impossible to overstate the importance of energy. Without it, we can do literally nothing. Further, the unfolding consequences of modern civilization's energy use (including climate change), together with the inevitable energy transition from fossil fuels to renewables, will be the defining trends of the current century. How we address the climate–energy dilemma will make a life-or-death difference for current and future generations of humans, and for countless other species.
But we can't participate usefully in discussions about energy without some basic knowledge of the subject. This chapter surveys a few simple energy concepts that everyone should be familiar with. These will include the definition of "energy" and an exploration of the forms it takes; the difference between energy and power; the Laws of Thermodynamics; the distinction between stocks and flows of energy; net energy (or energy returned on energy invested); lifecycle analysis (LCA) and lifecycle impacts; and the difference between operational energy and embodied energy.
What Is Energy? The Basics of the Basics
Energy is known by what it does: physicists define it as the capacity to do work. Energy exists in several forms — including thermal, radioactive decay, kinetic, mechanical, and electrical — and its form can change. For example, the energy stored in the molecular structures of coal can be released as heat through the process of combustion. That heat can be used to boil water, creating steam at high pressure, which can flow through turbines that spin magnets to produce an electric current, which is then passed through transformers and wires into homes and offices, where it is available to power computers, lights, and televisions.
Energy is measured in a variety of units, including joules, British thermal units (BTUs), and calories. When discussing electrical energy, the most common unit is the watt-hour (Wh). Sometimes the energy of fossil fuels is discussed in terms of barrels-of-oil-equivalent (boe). Where renewable energy is used in the form of electricity, we will discuss it in terms of watt-hours and megawatt-hours (MWh).
It is also important to understand the distinction between energy and power. While units of energy measure the total quantity of work done, they don't tell us how fast that work is being accomplished. For example, you could lift a one-ton boulder up the side of a mountain using only a small electric motor and a system of pulleys, but it would take a long time. A more powerful electric motor could do the job faster, while a still more powerful rocket engine could rapidly propel a payload of identical weight to the top of the mountain in a matter of seconds. Power is therefore defined as the rate at which energy is produced or used. Think of it as energy per unit of time. The standard unit of electrical power is the watt (W). The amount of electrical energy a 10 W light bulb uses depends on how long it is lit: in one hour, it will use 10 Wh of energy. In the same amount of time, a hundred thousand such bulbs would use 1000 kilowatt-hours (kWh), which equals 1 MWh (1,000,000 watts = 1000 kilowatts = 1 megawatt).
Laws of Thermodynamics
Two important physical principles, known as the first and second laws of thermodynamics, describe limits to the ways that energy works.
The first, known as the conservation law, states that energy cannot be created or destroyed, only transferred or transformed. In the example cited earlier (the use of coal to power household appliances), the total amount of energy is conserved at every stage. When energy chemically stored in the coal was transformed into heat, then electric current, and finally into the work of lighting our office or running our computer, some was "lost" at each stage. But that energy still exists; it is merely released to the environment, mostly as heat.
That's where the second law, sometimes called the entropy law, comes in: it states that, whenever energy is converted from one form to another, at least some of it is dissipated (again, typically as heat). Though that dissipated energy still exists, it is now diffuse and scattered and thus not available to do work. Thus, in effect, usable energy is always being lost. The word entropy was coined by the German physicist Rudolf Clausius in 1868 as a measure of the amount of energy no longer practically capable of conversion into work. According to the second law, the entropy within an isolated system inevitably increases over time. Since it takes work to create and maintain order within a system, the entropy law tells us the depressing news that, in the battle between order and chaos, it is chaos that ultimately will win.
All of this means that it is technically incorrect to say that we "consume" (or "produce") energy. We merely obtain it from places of higher concentration and get it to do work for us before it dissipates into less concentrated forms (ultimately, low-level dispersed heat). Hence energy density is an important criterion in assessing the likely value of potential energy resources (see the section "Energy Resource Criteria" later in the chapter). Only relatively concentrated energy is useful to us. We live in a universe teeming with energy; the trick is putting that energy to work. This is much easier when some of that energy happens to be temporarily concentrated in fossil fuels, wood, or uranium, or in a persistent flow pattern (e.g., a river, wind, or sunlight).
Areas of higher energy concentration can take either of two forms: stocks or flows. A stock is a store of energy — energy chemically stored in wood, oil, natural gas, or coal, or nuclear energy stored in stocks of uranium. Flows of energy include rivers, wind, and sunlight. Both stocks and flows present challenges: flows tend to be variable, whereas stocks can be depleted.
Some forms of energy are more versatile in their usefulness than others. For example, we can use electricity for a myriad of applications, whereas the heat from burning coal is currently used mostly for stationary applications like generating power (we formerly burned it in locomotives and ships, until oil proved its superiority for mobile applications). When we turn the heat from burning coal into electricity, a substantial amount of energy is lost due to the inefficiency of the process. But we are willing to accept that loss because coal is relatively cheap, and it would be difficult and inconvenient to use burning coal directly to power lights, computers, and refrigerators. In effect, we put a differing value on different forms of energy, with electricity at the top of the value ladder, liquid and gaseous fuels in the middle, and coal or firewood at the bottom. Solar and wind technologies have an advantage in that they produce high-value electricity directly.
Energy efficiency can be defined as minimizing the loss of energy in the process of obtaining work from an energy source. (How far can we get a gallon of gas to propel a two-ton automobile? That is the car's fuel efficiency, which is often expressed in miles per gallon). Efficiency also applies to converting energy from one form to another. (How much electricity can a power plant generate from a ton of coal or a thousand cubic feet of natural gas? Power plant efficiency is usually expressed as a percentage, indicating how much of the initial energy is still available after conversion).
Net Energy
It takes energy to get energy: for example, energy is needed to drill an oil well or build a solar panel. Only net energy, what is left over after our energy investment is subtracted, is actually useful to us for end-use purposes. Sometimes the relationship between energy investments and yields is expressed as a ratio, energy returned on energy invested (EROEI, or sometimes just EROI). For example, an EROEI of 10:1 indicates ten units of energy returned for every unit invested.
The historic economic bonanza resulting from society's use of fossil fuels partly ensued from the fact that, in the twentieth century, only trivial amounts of energy were required in drilling for oil or mining for coal as compared to the gush of energy yielded. High EROEI ratios (in the range of 100:1 or more) for society's energy-obtaining efforts meant that relatively small amounts of capital and labor were needed in order to supply all the energy that society could use. As a result, many people could be freed from basic energy-producing activities (like farming or forestry), their labor being substituted by fuel-fed machines. Channeled into manufacturing and managerial jobs, these people found ways to use abundant, cheap energy to produce ever more goods and services. The middle class mushroomed, as did cities and suburbs. In the process, we discovered an unintended consequence of having armies of cheap "energy slaves": as manufacturing and other sectors of the economy became mechanized, many handcraft professions disappeared.
The EROEI ratios for fossil fuels are declining as the best-quality resources are used up; meanwhile, the net energy figures of most renewable energy sources are relatively low compared to fossil fuels in their heyday (and this is especially true when buffering technologies — such as storage equipment and redundant capacity — are accounted for). A practical result of declining overall societal EROEI is the need to devote proportionally more capital and labor to energy production processes. This would likely translate, for example, to the requirement for more farm labor, and to fewer opportunities in professions not centered on directly productive activities: we would need more people making or growing things, and fewer people marketing, advertising, financing, regulating, and litigating them. For folks who think we have way too much marketing, advertising, financialization, regulation, and litigation in our current society, this might not seem like such a bad thing.
Net energy analysis (NEA) establishes a baseline for the economic usefulness of any energy resource. Decades of research suggest that, if an energy resource cannot yield at least three units of energy for every unit employed in energy production, it will not be economically useful in the long run unless the utilization of the energy is highly productive. A low net energy resource (such as biofuel) could potentially be of value if it provides a large benefit (e.g., as fuel for aviation if petroleum were to become scarce), but a high-EROEI resource would then be needed to provide the energy for the production of that lower-EROEI resource.
Unfortunately, the net energy or EROEI literature is inconsistent because researchers have so far been unable to agree on a common set of system boundaries. Therefore two analysts may calculate very different EROEI ratios for the same energy source. This does not entirely undermine the usefulness of NEA; it merely requires us to use caution in comparing the findings of different studies.
Incorporating the dimension of time into EROEI analysis adds yet another layer of complexity, but doing so is essential if we are to realistically compare energy from flows (solar and wind) with energy from stocks (fossil fuels). The great majority of the energy investment into solar panels comes during their manufacture, while the energy return is delivered slowly over the decades of their projected usefulness. This front-loading of energy investment creates problems if we wish to push the energy transition very quickly (as is documented in a study by Dale and Benson at Stanford University, who found that all solar technology installed until about 2010 was a net energy sink, in the sense that it hadn't yet paid for itself in energy terms). If solar and wind build-out rates are very high, the net energy available from these sources will be smaller during the transition period (though wind's higher EROEI should result in shorter delays in system-wide energy payback) (fig. 1.1). During at least the early stages of the transition, the kinds of energy being invested in building and deploying renewable energy systems (mostly fossil fuels for high-heat industrial purposes and for transportation) will be different from the higher-quality electrical energy yielded from those systems.
Life Cycle Impacts
Life cycle analysis (LCA) assesses the resource burden and potential environmental impacts associated with a product, process, or service by compiling an inventory of relevant energy and material inputs and environmental releases, and by evaluating the potential environmental impacts associated with identified inputs and releases. LCA is used to evaluate not just energy technologies but products and services of all kinds. However, since it typically tracks energy inputs (among other things), it is highly relevant for understanding our current energy systems and for planning the transition to renewables.
Virtually all energy processes entail environmental impacts, but some have greater impacts than others. These may occur during the acquisition of an energy resource (as in mining coal), or during the release of energy from the resource (as in burning wood, coal, oil, or natural gas), or in the conversion of the energy from one form to another (as in converting the kinetic energy of flowing water into electricity via a dam and hydro-turbines).
Some environmental impacts are indirect and occur in the manufacturing of the equipment used in energy harvesting or conversion. For example, the extraction and manipulation of resources used in manufacturing solar panels entail significantly more environmental damage than the operation of the panels themselves.
NEA and LCA are complementary: NEA ignores the environmental costs of energy production activities, whereas LCA identifies and quantifies these; on the other hand, LCA tells us nothing about the economic viability of an energy technology. LCA can be narrowed to encompass just materials use or energy use, or broadened to include greenhouse gas emissions and other environmental impacts. A shortcoming of LCA studies is that they represent a snapshot in time; for a process that hasn't changed much in decades (e.g., cement making) this is not a big issue, whereas for communications technology it means that studies can quickly become outdated.
LCA also gives us useful information about our energy-demand activities — manufacturing, transportation, building operation and maintenance, food production, communication, health care, and so on.
Operational versus Embodied Energy
Another essential energy concept has to do with the difference between embodied and operational energy. When we estimate the energy required by an automobile, for example, we are likely to think at first only of the gasoline in its tank. However, a substantial amount of energy was expended in the car's construction, in the mining of ores from which its metal components were made, in the manufacture of the mining equipment, and so on. Further, enormous amounts of energy were spent in building the infrastructure that enables us to use the car — including systems of roads and highways, and networks of service stations, refineries, pipelines, and oil wells. The gasoline in the car's fuel tank supplies operational energy, but much more energy is embodied in the car itself and its support systems (fig. 1.2). This latter energy expenditure is easily overlooked.
The energy glut of the twentieth century enabled us to embody energy in a mind-numbing array of buildings, roads, pipelines, machines, gadgets, and packaging. Middle-class families got used to buying and discarding enormous quantities of manufactured goods representing generous portions of previously expended energy. If we have less energy available to us in our renewable future, this may impact more than the operation of our machines and the lighting and heating of our buildings. It means those buildings, that infrastructure, and all those manufactured goods will be increasingly expensive to produce, which may translate to a shrinking flow of manufactured goods that embody past energy expenditure, and a reduced ability to construct high-energy-input structures. We might have to get used to consuming simpler foods again, rather than highly processed and excessively packaged ones. We might purchase, on average, fewer items of clothing and furniture and fewer electronic devices, and we might inhabit smaller spaces. We might use old goods longer, and reuse and repurpose whatever can be repaired. Exactly how far these trends might proceed is impossible to say. Nevertheless, under such conditions it is fair to assume that this overall shift might constitute the end of the network of economic arrangements collectively known as consumerism. Here again, there are more than a few people who believe that advanced industrial nations consume excessively, and that some simplification of upper- and middle-class lifestyles would be a good thing.
(Continues...)
Excerpted from Our Renewable Future by Richard Heinberg, David Fridley. Copyright © 2016 Post Carbon Institute. Excerpted by permission of ISLAND PRESS.
All rights reserved. No part of this excerpt may be reproduced or reprinted without permission in writing from the publisher.
Excerpts are provided by Dial-A-Book Inc. solely for the personal use of visitors to this web site.
Table of Contents
AcknowledgementsList of Figures and Tables
Introduction
-How “normal” came to be
-Why a renewable world will be different
-Overview of this book
PART I. The Context: It’s All About Energy
Chapter 1. Energy 101
-What is energy? The basics of the basics.
-Laws of Thermodynamics
-Net energy
-Lifecycle impacts
-Operational versus embodied energy
-Energy resource criteria
Chapter 2. A Quick Look At Our Current Energy System
-Growth
-Energy rich, energy poor
-Energy resources
-End use
PART II. Energy Supply in a Renewable World: Opportunities and Challenges
Chapter 3. Renewable Electricity: Falling Costs, Variability, and Scaling Challenges
-Price is less of a barrier
-Intermittency
-Storage
-Grid redesign
-Demand management
-Capacity redundancy
-Scaling challenges
-Lessons from Spain and Germany
-Pushback against wind and solar
Chapter 4. Transportation: The Substitution Challenge
-Electrification
-Biofuels
-Hydrogen
-Natural gas
-Sails and kites
-Summary: A less mobile all-renewable future
Chapter 5. Other Uses of Fossil Fuels: the Substitution Challenge Continues
-High temperature heat for industrial processes
-Low-temperature heat
-Fossil fuels for plastics, chemicals, and other materials
-Summary: Where’s our stuff?
Chapter 6. Energy Supply: How Much Will We Have? How Much Will We Need?
-EROEI of renewables
-Building solar and wind with solar and wind
-Investment requirements
-The efficiency opportunity: We may not need as much energy
-Energy Intensity
-The role of curtailment and the problem of economic growth
Chapter 7. What About…?
-Nuclear power
-Carbon capture and storage
-Massive technology improvements
PART III. Preparing For Our Renewable Future
Chapter 8. Energy and Justice
-Energy and equity in the least industrialized countries
-Energy and equity in rapidly industrializing nations
-Energy and equity in highly industrialized countries
-Policy frameworks for enhancing justice while cutting carbon
Chapter 9. What Government Can Do
-Support for an overall switch from fossil fuels to renewable energy
-Support for research and development of ways to use renewables to power more industrial processes and transport
-Conservation of fossil fuels for essential purposes
-Support for energy conservation in general—efficiency and curtailment
-Better greenhouse gas accounting
Chapter 10. What We the People Can Do
-Individuals and households
-Communities
-Climate and environmental groups, and their funders
Chapter 11. What We Learned
-We really need a plan; no, lots of them
-Scale is the biggest challenge
-It’s not all about solar and wind
-We must begin pre-adapting to having less energy
-Consumerism is a problem, not a solution
-Population growth makes everything harder
-Fossil fuels are too valuable to allocate solely by the market
-Everything is connected
-This really does change everything
About the Authors