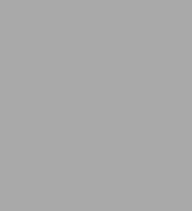
Photonic Crystals: Molding the Flow of Light (Second Edition)
304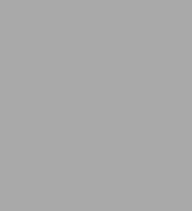
Photonic Crystals: Molding the Flow of Light (Second Edition)
304eBook
Related collections and offers
Overview
Since it was first published in 1995, Photonic Crystals has remained the definitive text for both undergraduates and researchers on photonic band-gap materials and their use in controlling the propagation of light. This newly expanded and revised edition covers the latest developments in the field, providing the most up-to-date, concise, and comprehensive book available on these novel materials and their applications.
Starting from Maxwell's equations and Fourier analysis, the authors develop the theoretical tools of photonics using principles of linear algebra and symmetry, emphasizing analogies with traditional solid-state physics and quantum theory. They then investigate the unique phenomena that take place within photonic crystals at defect sites and surfaces, from one to three dimensions. This new edition includes entirely new chapters describing important hybrid structures that use band gaps or periodicity only in some directions: periodic waveguides, photonic-crystal slabs, and photonic-crystal fibers. The authors demonstrate how the capabilities of photonic crystals to localize light can be put to work in devices such as filters and splitters. A new appendix provides an overview of computational methods for electromagnetism. Existing chapters have been considerably updated and expanded to include many new three-dimensional photonic crystals, an extensive tutorial on device design using temporal coupled-mode theory, discussions of diffraction and refraction at crystal interfaces, and more. Richly illustrated and accessibly written, Photonic Crystals is an indispensable resource for students and researchers.
- Extensively revised and expanded
- Features improved graphics throughout
- Includes new chapters on photonic-crystal fibers and combined index-and band-gap-guiding
- Provides an introduction to coupled-mode theory as a powerful tool for device design
- Covers many new topics, including omnidirectional reflection, anomalous refraction and diffraction, computational photonics, and much more.
Product Details
ISBN-13: | 9781400828241 |
---|---|
Publisher: | Princeton University Press |
Publication date: | 10/30/2011 |
Sold by: | Barnes & Noble |
Format: | eBook |
Pages: | 304 |
File size: | 23 MB |
Note: | This product may take a few minutes to download. |
About the Author
Read an Excerpt
Photonic Crystals
By John D. Joannopoulos Steven G. Johnson Joshua N. Winn Robert D. Meade Princeton University Press
Copyright © 2008 Princeton University Press
All right reserved.
ISBN: 978-0-691-12456-8
Chapter One Introduction
Controlling the Properties of Materials
Many of the true breakthroughs in our technology have resulted from a deeper understanding of the properties of materials. The rise of our ancestors from the Stone Age through the Iron Age is largely a story of humanity's increasing recognition of the utility of natural materials. Prehistoric people fashioned tools based on their knowledge of the durability of stone and the hardness of iron. In each case, humankind learned to extract a material from the Earth whose fixed properties proved useful.
Eventually, early engineers learned to do more than just take what the Earth provides in raw form. By tinkering with existing materials, they produced substances with even more desirable properties, from the luster of early bronze alloys to the reliability of modern steel and concrete. Today we boast a collection of wholly artificial materials with a tremendous range of mechanical properties, thanks to advances in metallurgy, ceramics, and plastics.
In this century, our control over materials has spread to include their electrical properties. Advances in semiconductor physics have allowed us to tailor the conducting properties of certain materials, thereby initiating the transistorrevolution in electronics. It is hard to overstate the impact that the advances in these fields have had on our society. With new alloys and ceramics, scientists have invented high-temperature superconductors and other exotic materials that may form the basis of future technologies.
In the last few decades, a new frontier has opened up. The goal in this case is to control the optical properties of materials. An enormous range of technological developments would become possible if we could engineer materials that respond to light waves over a desired range of frequencies by perfectly reflecting them, or allowing them to propagate only in certain directions, or confining them within a specified volume. Already, fiber-optic cables, which simply guide light, have revolutionized the telecommunications industry. Laser engineering, high-speed computing, and spectroscopy are just a few of the fields next in line to reap the benefits from the advances in optical materials. It is with these goals in mind that this book is written.
Photonic Crystals
What sort of material can afford us complete control over light propagation? To answer this question, we rely on an analogy with our successful electronic materials. A crystal is a periodic arrangement of atoms or molecules. The pattern with which the atoms or molecules are repeated in space is the crystal lattice. The crystal presents a periodic potential to an electron propagating through it, and both the constituents of the crystal and the geometry of the lattice dictate the conduction properties of the crystal.
The theory of quantum mechanics in a periodic potential explains what was once a great mystery of physics: In a conducting crystal, why do electrons propagate like a diffuse gas of free particles? How do they avoid scattering from the constituents of the crystal lattice? The answer is that electrons propagate as waves, and waves that meet certain criteria can travel through a periodic potential without scattering (although they will be scattered by defects and impurities).
Importantly, however, the lattice can also prohibit the propagation of certain waves. There may be gaps in the energy band structure of the crystal, meaning that electrons are forbidden to propagate with certain energies in certain directions. If the lattice potential is strong enough, the gap can extend to cover all possible propagation directions, resulting in a complete band gap. For example, a semiconductor has a complete band gap between the valence and conduction energy bands.
The optical analogue is the photonic crystal, in which the atoms or molecules are replaced by macroscopic media with differing dielectric constants, and the periodic potential is replaced by a periodic dielectric function (or, equivalently, a periodic index of refraction). If the dielectric constants of the materials in the crystal are sufficiently different, and if the absorption of light by the materials is minimal, then the refractions and reflections of light from all of the various interfaces can produce many of the same phenomena for photons (light modes) that the atomic potential produces for electrons. One solution to the problem of optical control and manipulation is thus a photonic crystal, a low-loss periodic dielectric medium. In particular, we can design and construct photonic crystals with photonic band gaps, preventing light from propagating in certain directions with specified frequencies (i.e., a certain range of wavelengths, or "colors," of light). We will also see that a photonic crystal can allow propagation in anomalous and useful ways.
To develop this concept further, consider how metallic waveguides and cavities relate to photonic crystals. Metallic waveguides and cavities are widely used to control microwave propagation. The walls of a metallic cavity prohibit the propagation of electromagnetic waves with frequencies below a certain threshold frequency, and a metallic waveguide allows propagation only along its axis. It would be extremely useful to have these same capabilities for electromagnetic waves with frequencies outside the microwave regime, such as visible light. However, visible light energy is quickly dissipated within metallic components, which makes this method of optical control impossible to generalize. Photonic crystals allow the useful properties of cavities and waveguides to be generalized and scaled to encompass a wider range of frequencies. We may construct a photonic crystal of a given geometry with millimeter dimensions for microwave control, or with micron dimensions for infrared control.
Another widely used optical device is a multilayer dielectric mirror, such as a quarter-wave stack, consisting of alternating layers of material with different dielectric constants. Light of the proper wavelength, when incident on such a layered material, is completely reflected. The reason is that the light wave is partially reflected at each layer interface and, if the spacing is periodic, the multiple reflections of the incident wave interfere destructively to eliminate the forward-propagating wave. This well-known phenomenon, first explained by Lord Rayleigh in 1887, is the basis of many devices, including dielectric mirrors, dielectric Fabry-Perot filters, and distributed feedback lasers. All contain low-loss dielectrics that are periodic in one dimension, and by our definition they are one-dimensional photonic crystals. Even these simplest of photonic crystals can have surprising properties. We will see that layered media can be designed to reflect light that is incident from any angle, with any polarization-an omnidirectional reflector-despite the common intuition that reflection can be arranged only for near-normal incidence.
If, for some frequency range, a photonic crystal prohibits the propagation of electromagnetic waves of any polarization traveling in any direction from any source, we say that the crystal has a complete photonic band gap. A crystal with a complete band gap will obviously be an omnidirectional reflector, but the converse is not necessarily true. As we shall see, the layered dielectric medium mentioned above, which cannot have a complete gap (because material interfaces occur only along one axis), can still be designed to exhibit omnidirectional reflection-but only for light sources far from the crystal. Usually, in order to create a complete photonic band gap, one must arrange for the dielectric lattice to be periodic along three axes, constituting a three-dimensional photonic crystal. However, there are exceptions. A small amount of disorder in an otherwise periodic medium will not destroy a band gap (Fan et al., 1995b; Rodriguez et al., 2005), and even a highly disordered medium can prevent propagation in a useful way through the mechanism of Anderson localization (John, 1984). Another interesting nonperiodic class of materials that can have complete photonic band gaps are quasi-crystalline structures (Chan et al., 1998).
An Overview of the Text
Our goal in writing this textbook was to provide a comprehensive description of the propagation of light in photonic crystals. We discuss the properties of photonic crystals of gradually increasing complexity, beginning with the simplest case of one-dimensional crystals, and proceeding to the more intricate and useful properties of two-and three-dimensional systems (see figure 1). After equipping ourselves with the appropriate theoretical tools, we attempt to convey a useful intuition about which structures yield what properties, and why?
This textbook is designed for a broad audience. The only prerequisites are a familiarity with the macroscopic Maxwell equations and the notion of harmonic modes (which are often referred to by other names, such as eigenmodes, normal modes, and Fourier modes). From these building blocks, we develop all of the needed mathematical and physical tools. We hope that interested undergraduates will find the text approachable, and that professional researchers will find our heuristics and results to be useful in designing photonic crystals for their own applications.
Readers who are familiar with quantum mechanics and solid-state physics are at some advantage, because our formalism owes a great deal to the techniques and nomenclature of those fields. Appendix A explores this analogy in detail. Photonic crystals are a marriage of solid-state physics and electromagnetism. Crystal structures are citizens of solid- state physics, but in photonic crystals the electrons are replaced by electromagnetic waves. Accordingly, we present the basic concepts of both subjects before launching into an analysis of photonic crystals. In chapter 2, we discuss the macroscopic Maxwell equations as they apply to dielectric media. These equations are cast as a single Hermitian differential equation, a form in which many useful properties become easy to demonstrate: the orthogonality of modes, the electromagnetic variational theorem, and the scaling laws of dielectric systems.
Chapter 3 presents some basic concepts of solid-state physics and symmetry theory as they apply to photonic crystals. It is common to apply symmetry arguments to understand the propagation of electrons in a periodic crystal potential. Similar arguments also apply to the case of light propagating in a photonic crystal. We examine the consequences of translational, rotational, mirror-reflection, inversion, and time-reversal symmetries in photonic crystals, while introducing some terminology from solid-state physics.
To develop the basic notions underlying photonic crystals, we begin by reviewing the properties of one-dimensional photonic crystals. In chapter 4, we will see that one-dimensional systems can exhibit three important phenomena: photonic band gaps, localized modes, and surface states. Because the index contrast is only along one direction, the band gaps and the bound states are limited to that direction. Nevertheless, this simple and traditional system illustrates most of the physical features of the more complex two-and three-dimensional photonic crystals, and can even exhibit omnidirectional reflection.
In chapter 5, we discuss the properties of two-dimensional photonic crystals, which are periodic in two directions and homogeneous in the third. These systems can have a photonic band gap in the plane of periodicity. By analyzing field patterns of some electromagnetic modes in different crystals, we gain insight into the nature of band gaps in complex periodic media. We will see that defects in such two-dimensional crystals can localize modes in the plane, and that the faces of the crystal can support surface states.
Chapter 6 addresses three-dimensional photonic crystals, which are periodic along three axes. It is a remarkable fact that such a system can have a complete photonic band gap, so that no propagating states are allowed in any direction in the crystal. The discovery of particular dielectric structures that possess a complete photonic band gap was one of the most important achievements in this field. These crystals are sufficiently complex to allow localization of light at point defects and propagation along linear defects.
Chapters 7 and 8 consider hybrid structures that combine band gaps in one or two directions with index-guiding (a generalization of total internal reflection) in the other directions. Such structures approximate the three-dimensional control over light that is afforded by a complete three-dimensional band gap, but at the same time are much easier to fabricate. Chapter 9 describes a different kind of incomplete-gap structure, photonic-crystal fibers, which use band gaps or index-guiding from one-or two-dimensional periodicity to guide light along an optical fiber.
Finally, in chapter 10, we use the tools and ideas that were introduced in previous chapters to design some simple optical components. Specifically, we see how resonant cavities and waveguides can be combined to form filters, bends, splitters, nonlinear "transistors," and other devices. In doing so, we develop a powerful analytical framework known as temporal coupled-mode theory, which allows us to easily predict the behavior of such combinations. We also examine the reflection and refraction phenomena that occur when light strikes an interface of a photonic crystal. These examples not only illustrate the device applications of photonic crystals, but also provide a brief review of the material contained elsewhere in the text.
We should also mention the appendices, which provide a brief overview of the reciprocal-lattice concept from solid-state physics, survey the gaps that arise in various two-and three-dimensional photonic crystals, and outline the numerical methods that are available for computer simulations of photonic structures.
(Continues...)
Excerpted from Photonic Crystals by John D. Joannopoulos Steven G. Johnson Joshua N. Winn Robert D. Meade
Copyright © 2008 by Princeton University Press. Excerpted by permission.
All rights reserved. No part of this excerpt may be reproduced or reprinted without permission in writing from the publisher.
Excerpts are provided by Dial-A-Book Inc. solely for the personal use of visitors to this web site.
Table of Contents
Preface to the Second Edition xiii
Preface to the First Edition xv
Chapter 1: Introduction 1
Controlling the Properties of Materials 1
Photonic Crystals 2
An Overview of the Text 3
Chapter 2: Electromagnetism in Mixed Dielectric Media 6
The Macroscopic Maxwell Equations 6
Electromagnetism as an Eigenvalue Problem 10
General Properties of the Harmonic Modes 12
Electromagnetic Energy and the Variational Principle 14
Magnetic vs. Electric Fields 16
The Effect of Small Perturbations 17
Scaling Properties of the Maxwell Equations 20
Discrete vs. Continuous Frequency Ranges 21
Electrodynamics and Quantum Mechanics Compared 22
Further Reading 24
Chapter 3: Symmetries and Solid-State Electromagnetism 25
Using Symmetries to Classify Electromagnetic Modes 25
Continuous Translational Symmetry 27
Index guiding 30
Discrete Translational Symmetry 32
Photonic Band Structures 35
Rotational Symmetry and the Irreducible Brillouin Zone 36
Mirror Symmetry and the Separation of Modes 37
Time-Reversal Invariance 39
Bloch-Wave Propagation Velocity 40
Electrodynamics vs. Quantum Mechanics Again 42
Further Reading 43
Chapter 4: The Multilayer Film: A One-Dimensional Photonic Crystal 44
The Multilayer Film 44
The Physical Origin of Photonic Band Gaps 46
The Size of the Band Gap 49
Evanescent Modes in Photonic Band Gaps 52
Off-Axis Propagation 54
Localized Modes at Defects 58
Surface States 60
Omnidirectional Multilayer Mirrrors 61
Further Reading 65
Chapter 5: Two-Dimensional Photonic Crystals 66
Two-Dimensional Bloch States 66
A Square Lattice of Dielectric Columns 68
A Square Lattice of Dielectric Veins 72
A Complete Band Gap for All Polarizations 74
Out-of-Plane Propagation 75
Localization of Light by Point Defects 78
Point defects in a larger gap 83
Linear Defects and Waveguides 86
Surface States 89
Further Reading 92
Chapter 6: Three-Dimensional Photonic Crystals 94
Three-Dimensional Lattices 94
Crystals with Complete Band Gaps 96
Spheres in a diamond lattice 97
Yablonovite 99
The woodpile crystal 100
Inverse opals 103
A stack of two-dimensional crystals 105
Localization at a Point Defect 109
Experimental defect modes in Yablonovite 113
Localization at a Linear Defect 114
Localization at the Surface 116
Further Reading 121
Chapter 7: Periodic Dielectric Waveguides 122
Overview 122
A Two-Dimensional Model 123
Periodic Dielectric Waveguides in Three Dimensions 127
Symmetry and Polarization 127
Point Defects in Periodic Dielectric Waveguides 130
Quality Factors of Lossy Cavities 131
Further Reading 134
Chapter 8: Photonic-Crystal Slabs 135
Rod and Hole Slabs 135
Polarization and Slab Thickness 137
Linear Defects in Slabs 139
Reduced-radius rods 139
Removed holes 142
Substrates, dispersion, and loss 144
Point Defects in Slabs 147
Mechanisms for High Q with Incomplete Gaps 149
Delocalization 149
Cancellation 151
Further Reading 155
Chapter 9: Photonic-Crystal Fibers 156
Mechanisms of Confinement 156
Index-Guiding Photonic-Crystal Fibers 158
Endlessly single-mode fibers 161
The scalar limit and LP modes 163
Enhancement of nonlinear effects 166
Band-Gap Guidance in Holey Fibers 169
Origin of the band gap in holey fibres 169
Guided modes in a hollow core 172
Bragg Fibers 175
Analysis of cylindrical fibers 176
Band gaps of Bragg fibers 178
Guided modes of Bragg fibers 180
Losses in Hollow-Core Fibers 182
Cladding losses 183
Inter-modal coupling 187
Further Reading 189
Chapter 10: Designing Photonic Crystals for Applications 190
Overview 190
A Mirror, a Waveguide, and a Cavity 191
Designing a mirror 191
Designing a waveguide 193
Designing a cavity 195
A Narrow-Band Filter 196
Temporal Coupled-Mode Theory 198
The temporal coupled-mode equations 199
The filter transmission 202
A Waveguide Bend 203
A Waveguide Splitter 206
A Three-Dimensional Filter with Losses 208
Resonant Absorption and Radiation 212
Nonlinear Filters and Bistability 214
Some Other Possibilities 218
Reflection, Refraction, and Diffraction 221
Reflection 222
Refraction and isofrequency diagrams 223
Unusual refraction and diffraction effects 225
Further Reading 228
Epilogue 228
A Comparisons with Quantum Mechanics 229
B The Reciprocal Lattice and the Brillouin Zone 233
The Reciprocal Lattice 233
Constructing the Reciprocal Lattice Vectors 234
The Brillouin Zone 235
Two-Dimensional Lattices 236
Three-Dimensional Lattices 238
Miller Indices 239
C Atlas of Band Gaps 242
A Guided Tour of Two-Dimensional Gaps 243
Three-Dimensional Gaps 251
D Computational Photonics 252
Generalities 253
Frequency-Domain Eigenproblems 255
Frequency-Domain Responses 258
Time-Domain Simulations 259
A Planewave Eigensolver 261
Further Reading and Free Software 263
Bibliography 265
Index 283
What People are Saying About This
An excellent textbook to be used in physics, chemistry, and engineering. The revised edition of Photonic Crystals fills the gap between the layperson and the expert reader.
Costas M. Soukoulis, Iowa State University
This book is destined to become the classic textbook in the area. It gathers together the fundamental concepts and tools relevant to photonic crystals and presents them with exceptional clarity. I genuinely enjoyed reading it.
Maryanne Large, University of Sydney